Once the stuff of science fiction, the International Space Station (ISS) offers a unique test-bed for physics in microgravity conditions. Moreover, describes Bruce Dorminey, experiments carried out on the ISS are paving the way for manned missions to Mars and beyond
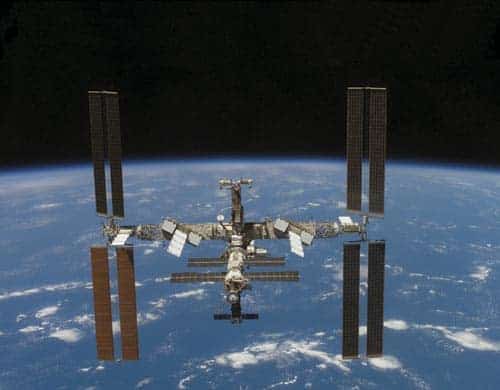
Exponentially over budget, plagued by technical glitches and some seven years behind schedule, critics have always found the International Space Station (ISS) to be an easy target. Since NASA first began discussing the station’s forerunner some 25 years ago, many astrophysicists and planetary scientists have viewed the ISS as an orbiting “white elephant” siphoning funds from more scientifically adventurous space missions.
But that would be to ignore the importance of having a permanently manned space station. While the ISS’s interlocking modules, external trusses and solar arrays hardly resemble Arthur C Clarke’s majestic rotating wheel in 2001: A Space Odyssey, the station is a product of humankind’s quest for both a better life here on Earth and an innate sense of wanderlust. Born a quarter of a century after the Soviet Union launched Sputnik 1, the ISS grew out of an amalgam of designs from previously planned but unexecuted space stations. These include the US Space Station Freedom, Russia’s Mir-2 and the stand-alone Columbus research module of the European Space Agency (ESA).
Today, as a joint project of the US, Russian, European, Japanese and Canadian space agencies, the ISS orbits at an altitude of between 370–460 km in the same direction as Earth’s rotation. It provides a unique environment in which to study nature in low gravity — from the flow of fluids to the growth of crystal. Moreover, the ISS is proving to be a “research springboard” from which humankind can launch itself further out into the solar system. That is, if more down-to-earth factors such as money and international politics do not get in the way.
A political science
From the outset, critics of US space policy singled out the ISS as a foray into post-Cold War politics, rather than truly an international scientific undertaking. As planetary scientist Wendell Mendell at NASA’s Johnson Space Center in Houston explains, “The NASA space programme is a technically driven enterprise intended to explore the unknown. But because it is part of the US government, it is also a political entity. Except for the fact that NASA wanted to build one, in my mind the space station was not well thought out. It was a committee-consensus process, so over the years there were a lot of studies that ended up getting scrapped.”
Once the ISS reaches the “assembly complete” stage by 2010 — which was originally scheduled for 2003 — a permanent crew of six will enjoy a pressurized volume of 935 m3 during six-month-long stays. That is about four times larger than the Russian Mir space station, which was manned continuously for almost 10 years before being forced to crash into the atmosphere in 2001, and some five times the size of NASA’s 1970s-era Skylab. Unofficially, NASA’s expenditure on the ISS is $100bn and counting — spiking through its years of planning and construction. Even so, ISS funding is assured until 2016, and it is likely that the station will operate until at least 2020.
In late 1998 Russia delivered the first segment of the ISS to low Earth orbit, and for a while the assembly appeared to be running on schedule. But with the loss in 2003 of the Space Shuttle Columbia and its crew of seven, the US Congress argued that if astronauts were willing to risk their lives for spaceflight, then NASA needed to make sure that the risk was commensurate with the exploratory and scientific gain. While the ISS might have had a noble purpose, was it really pushing the bounds of exploration in the tradition of, say, the Apollo programme that took us to the Moon nearly 40 years ago?
Even though the aerospace industry seemed excited about the ISS, many manned-spaceflight enthusiasts — ranging from researchers within the space agencies themselves to space buffs among the general public — viewed it as a distraction from more scientifically daring missions. Thus, if the ISS itself was not really pushing the boundaries, then its international partners had two choices: either abandon it altogether; or persevere and use it as jumping-off point for research on how to achieve long-term manned lunar and interplanetary missions. Fortunately they chose the latter option.
High dining
The ISS can tell us a lot about manned-spaceflight operations but, as Mendell points out, the devil is in the details. “Nowadays there is a lot of talk about the problems of garbage and stowage because no-one had thought about those before,” he says. “The whole idea of how humans perform in isolated conditions in space for long durations is an important element of study in the ISS.”
A case in point: after the ISS astronauts have dined on some of their favourite in-orbit offerings — which include shrimp cocktail, chicken fajitas and barbecue-beef brisket all washed down with lemonade — ground control requires that they spend at least 1.5 hours doing resistive exercise. As well as keeping the astronauts’ heart muscles functioning optimally, the workout counters the bone loss that humans suffer in microgravity conditions (i.e. a state where the force of gravity is almost undetectable and which is practically the same as weightlessness). In such a low-gravity environment, astronauts can lose up to 2% of their bone structure per month. No-one knows exactly why this happens, but it is thought that a lack of gravitational stress on the skeletal structure somehow slows production of bonebuilding osteoblast cells.
If manned trips to Mars are to be feasible, then space biologists are going to have to solve some very basic problems associated with long-term weightlessness. Thus, during their regular five days per week work schedule, crew members on the ISS spend much of their time helping ground-based investigators carry out hundreds of microgravity experiments.
About 200 experiments have already been carried out on the space station or are still in progress, and at least 500 more are planned over the next five years. They range from Earth observations to proving the worth of technologies for industry, including many that study the effect of microgravity on animal, plant and human biology. Thus far, these experiments have been carried out in the Russian service modules and the US research lab Destiny. However, the Space Shuttle is scheduled to deploy ESA’s Columbus laboratory in December of this year and the Japanese Kibo research module in April 2008. Furthermore, the Russians hope to develop and deploy a research module perhaps as early as 2011.
Each ISS partner is responsible for choosing (and funding) its own experiments, which usually begins with some sort of peer-review process to weigh up the merits of individual scientific proposals. It can take anywhere from six months to eight years before these proposals are finally implemented in Earth orbit. While most of the experiments do not require much involvement from astronauts, the crew members often have to start and stop experiments as well as to document results using digital imagery and video for later ground-based analysis. But given that many of the astronauts already have PhDs in a science subject, they are usually very comfortable with the rigours of experimental investigation.
Fuelled by experiment
Recently, much of the focus of ISS experiments has been to refine technologies that will help humankind explore beyond the Moon to Mars, such as those that manage spacecraft fuels. “Right after Sputnik, it dawned on NASA that when gravity goes, liquid fuels are also going to do different things,” says Mark Weislogel, a mechanical engineer at Portland State University in Oregon. “The Apollo engineers implemented their designs without the benefit of long-duration microgravity tests. They made a series of good decisions, but they had a measure of luck on their side. With more low-gravity experience, however, we can improve the reliability of systems and reduce the overall mass of a given spacecraft.”
Understanding how fluids behave in the absence of gravity is vital when managing spacecraft fuel tanks. But it is also important for life-support systems, liquidwaste disposal, water processing, thermal cooling and potentially even space-based turbine-powered electrical generators. In 2004 Weislogel was the principal investigator on a series of capillary-flow experiments on the ISS, in which he and his co-workers investigated how capillary surface-tension forces drive fluids both in space and on the ground. To study capillary forces in space, ISS astronauts used digitized video to record the movements of silicone oil contained in six 2 kg test vessels. The data are currently being analysed by researchers back on Earth.
“Suppose you’ve just released one of your rocket’s upper stages and now you’re adrift in low gravity,” says Weislogel. “It’s time to fire the next rocket, but if the tank isn’t full, you’ve got to know where that liquid is. If the engine fires and the liquid isn’t over the exit, then you could have a problem.” In other words, if microgravity changes the location of fuel in the tank, which in some spacecraft designs has to be mixed in precise ratios from two separate fuel components, the engines may misfire and the fuel tanks could even be damaged. Such scenarios could cause a spacecraft to miss its target, with potentially disastrous consequences.
Fuel tanks tend to be spheroidal in order to make them as strong as possible. But even with the best current designs, low gravity can play havoc when it comes to positioning fuel within a tank. To get round the problem, designers frequently use complicated propellantmanagement devices or baffles to wick the fuel into its optimal position, which is usually near a fuel pump. However, if an Apollo-era device failed, the engine might be knocked out altogether — possibly interfering with the mission’s ability to manoeuvre back safely to Earth. Weislogel and co-workers’ capillary-flow experiments could reduce such risks. For instance, if the primary fuel system fails, better use of capillary forces could ensure that at least some of the spacecraft’s cooling or other systems might still function, even if at a reduced level.
If faulty fuel pumps do not end a mission altogether, there is always the ongoing threat to astronauts from solar flares made up of very energetic protons or of heavy-ion background radiation from galactic cosmic rays (GCRs). In particular, the highly ionizing nature of GCRs can cause proteins in human cells to fragment, which increases the chances of tissue damage and tumours. While the Earth’s magnetic field protects ISS crews from much of the Sun’s activity, this will not be the case for manned interplanetary missions.
Frank Cucinotta, a radiation biologist and chief scientist for NASA’s Radiation Research Program at the Johnson Space Center, says that we have come a long way in our understanding of radiation risks since the days of Yuri Gagarin, who in 1961 became the first man in space. “We now understand how radiation traverses through the materials [both of the spacecraft itself and the astronauts’ attire] and tissue,” he says. “By studying the damage mechanism, we should be able to develop biological countermeasures such as antioxidants, pharmaceuticals and gene therapy.”
The ISS experiment Matroshka-2 (MTR-2), which follows on from an earlier experiment called Matroshka-1, was designed to track radiation fluxes both inside and outside the space station. MTR-2 uses a simulated human torso to mimic human flesh and internal organs. This “phantom” is embedded with dosimeters to measure incoming radiation fluxes, which can then be compared with the latest space-radiation models to better estimate the real risk to humans.
MTR-2’s principal investigator Guenther Reitz, who heads the department of radiation biology at the German Aerospace Center in Cologne, says fluxes of cosmic-ray exposure outside the ISS have been “overestimated” in the past. He and his colleagues, who are currently working on a paper summarizing their findings for Nature, are encouraged by their initial results. While all humans have different sensitivities to radiation, Reitz says there may be ways, however futuristic, to capitalize on radiation-resistant genetic traits to help make astronauts less susceptible to ambient radiation in space. He says that cosmic rays are a high risk, but they will not stop humans from making interstellar trips beyond our solar system’s heliopause.
Breaking the second law
Although the ISS has been a boon for research into the biological impact of life in low Earth orbit, the microgravity conditions on board the station have also provided an important niche for fluids and materials research. One such experiment explores one of the most basic tenets of high-school physics: the second law of thermodynamics, which states that entropy (a measure of disorder) always increases when a system changes from one state to another.
When a crystal forms in microgravity, the individual particles in a system have more freedom to rattle about than they would on the ground. Thus, in space an ordered structure can, somewhat perversely, arise from a higher state of entropy. “Take colloids [microparticles suspended in liquid] into space,” says William Meyer, a staff scientist at the National Center for Fluids and Combustion at NASA’s Glenn Research Center in Cleveland, “and you automatically take away the sedimentation and jamming effect of gravity.”
This can have striking consequences. As principal investigator for the Express Physics of Colloids in Space experiment, in 2004 physicist David Weitz of Harvard University and his colleagues used colloidal engineering to study the microgravity dynamics of polymethyl methacrylate — a particle form of Plexiglass — suspended in an organic solvent. They found that more ordered and larger liquid-crystal-type structures can form on the ISS than on Earth because particles can remain suspended indefinitely in microgravity. This, in turn, leads to crystals that can diffract light more effectively, possibly leading to “perfect mirrors”.
An obvious Earth-bound application of a perfect mirror is in fibre-optic telecommunications. For example, if a perfect-mirror coating could be developed, it could prevent signal loss in fibre-optic cables, particularly when such signals (i.e. light) are forced to make sharp turns. Currently, fibre-optic switching is performed electronically by first converting light into electricity, processing the signal and then converting it back into photons again. But according to Meyer, such devices — for example based on a “photonic-band-gap mirror” — would allow the same amount of light to handle a lot more information, thus potentially making fibre-optic communications much more efficient.
A less hi-tech, but perhaps more surprising, application of Weitz and co-workers’ Plexiglass experiments might be found in detergent products. If detergent and even some food manufacturers had a better understanding of a given product’s shelf life (which is often governed by its rate of gravitational collapse), such knowledge could potentially save them millions of dollars. For example, Weitz says that at least one major US detergent manufacturer would like to make its current fabric-softener formulation more polymer-rich so that it makes clothes feel softer. But a higher polymer count can sometimes translate into a less stable product. Thus, one goal of Weitz’ ISS research is to simply determine how to avoid product instabilities, while extending a given product’s shelf life.
But Weitz is also interested in making materials that can survive long periods in low gravity — materials that will be needed to assure successful interplanetary missions. “We mixed these colloidal particles with some polymer and saw behaviour analogous to that of oil and water in salad dressing,” he says. “That helps us understand the stability of common everyday products and why things remain stable. If we’re serious about going to Mars, we had better understand if things that normally remain stable on Earth also remain stable in space.”
From detergents to Mars
Ironically, the long journey from Earth orbit to Mars may finally begin when the Space Shuttle is retired in 2010. It is due to be replaced by NASA’s Crew Exploration Vehicle (CEV), which is now scheduled for a 2014 launch via NASA’s planned Ares I rocket. The CEV (see over) will be able to ferry a crew of six to the ISS and back. And both the CEV and ESA’s Automated Transfer Vehicle (ATV) will be used to autonomously replenish station cargoes. Currently, Russia’s unpiloted Progress spacecraft functions as an ISS resupply vehicle, while Japan is also planning a robotic resupply spacecraft — the H-II Transfer Vehicle (HTV) — for launch in 2009. Later, NASA also plans to use the CEV to take astronauts back to the Moon — a mission now projected for 2020 — with a mission to Mars within a few years.
NASA’s original Apollo schedule called for a lunar base by the mid- to late-1970s. Who would have predicted that 30 years hence, the US agency would be struggling to remount technology just to get back to the lunar surface? If the red planet remains NASA’s real goal, however, the ISS will continue to pay intangible dividends simply in terms of learning more about the vagaries of long-duration spaceflight.
For example, astronauts on their way to Mars will need to be more autonomous and resourceful because ground controllers will not be able to monitor and supervise them in real time. “It will be more like communications at the South Pole in the age of the Telex,” says Mendell. “The missions will still need back-room support, but we’ve got to figure out how to do it differently.” The ISS is already helping long-term planners tackle such practical issues, but it is doing so from Earth orbit.
Half a century after the launch of Sputnik 1, what can we say of the ISS and of the future human occupation of space? Arthur C Clarke’s iconic year 2001 has long since passed, without having delivered Stanley Kubrick’s grand cinematic vision of ambitious interplanetary missions and routine trips to the lunar surface. Yet even if humanity stands guilty of squandering its past glories due to a sometimes-indolent space policy, we can be thankful that at least new chapters in the history of manned spaceflight continue to be written. We are now using the ISS to plan our next steps out into the unknown. With the Moon as a way station, first stop will be Mars. And for its role in teaching us how to get there and back, the ISS is proving to be serendipity indeed.