Michael Riordan continues his look back on the Higgs boson search with the early attempts to hunt it down at the Tevatron and the Large Hadron Collider
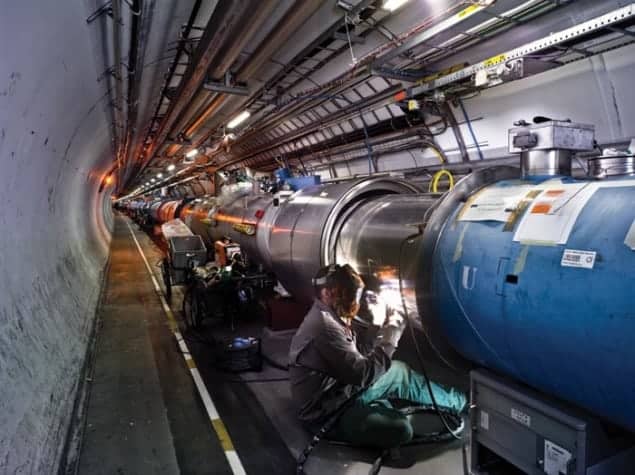
In one sense, the Large Electron–Positron (LEP) collider at CERN could have been considered a failure. Although LEP had cost about a billion Swiss francs (CHF) to build, and even more than that to operate from 1989 to 2000, researchers did not discover a single new elementary particle using it. Sure, they made tremendous refinements of the properties of the massive W and Z bosons – the weak-force-bearing particles that had been discovered at CERN in the early 1980s – as well as precision measurements of other important parameters of the Standard Model of particle physics. But during that 12-year period, only Fermilab could claim a fundamental particle discovery – of the top quark in 1995.
In another sense, however, LEP was a major success. For physicists had excluded a huge range of masses in which any Higgs bosons would have been almost impossible to discover by experiments at proton colliders. A particle that remotely resembled the Higgs boson predicted by the Standard Model should have appeared in electron-positron collisions at LEP had its mass been up to as high as 114 billion electron volts (114 GeV), according to a combined analysis of the four LEP experiments published in 2003 (Phys. Lett. B 565 61). But nothing new had cropped up in this range. And the precision LEP measurements, taken together with the top-quark mass as determined at Fermilab, required that any Standard Model Higgs boson had to show up at a mass below 193 GeV (at a confidence level of 95%). As nobody could have said anything much about its mass before 1989, LEP researchers had thus taken a giant step on the long road to cornering the Higgs boson.
And many physicists on the ALEPH experiment at LEP, which had recorded the most telling candidate events, argued that they had witnessed good evidence for it at 115 GeV. In December 2000 they published a paper entitled “Observation of an excess in the search for the Standard Model Higgs boson at ALEPH” (Phys. Lett. B 495 1), claiming a 3σ excess of Higgs-like events at this energy. But the other three LEP experiments did not confirm these results. Therefore the combined analysis allowed only that such a signal might have occurred – in other words, the signal-plus-background hypothesis fitted all the data better than no signal at all, but not by much. In late 2000 CERN finally shut LEP down after a heated debate and began construction of the Large Hadron Collider (LHC).
Focus on Fermilab
As the new century dawned, physicists at Fermilab could look forward to more than five fruitful years during which they had no competition at all in the Higgs search. It would take at least that long (and as it turned out, much longer) to build and install the LHC in the 27?km LEP tunnel. Boasting a collision energy of almost 2 × 1012 eV (2 TeV), Fermilab’s Tevatron proton–antiproton collider was then the most powerful machine on Earth – and the only one able to generate exotic new particles with masses above 100 GeV. But would it have a sufficiently high collision rate, or luminosity, to create enough of the expectedly rare Higgs events?
A daunting problem with hadron colliders such as the Tevatron or the LHC is that they also produce lots of extraneous debris because protons and antiprotons are not elementary but composite particles made of quarks and gluons. Indeed, Caltech theorist Richard Feynman once compared proton collisions to “smashing garbage cans into garbage cans”. A lot of garbage comes bursting out, some of it looking a lot like the expected decay products of Higgs bosons. At LEP this was not a problem because it collided electrons and positrons, which are essentially point particles with well-understood electromagnetic interactions. Its candidate Higgs events had only two or four tightly packed “jets” of hadrons, corresponding to emerging quarks, and little else. These events could be recognized rather easily.
But at the Tevatron and the LHC, such Higgs-like signals would be swamped by the immense backgrounds of ordinary hadron events. It is not unlike trying to detect a fire-fighter smoking a cigarette in the midst of a forest fire from the two different smoke patterns emitted. If you happen to have a strong, distinctive signal, digging it out from such backgrounds is easier. But if not, experimenters must try to accumulate a tremendous number of events to be certain they can convincingly extract a meaningful signal from the smothering background. And that takes luminosity or time. Or both.
At the lower masses where the Higgs boson was thought most likely to lurk after the LEP shutdown, from 115 GeV to 193 GeV, there was one possible strong, distinctive signal – at just above 155 GeV, where a Standard Model Higgs boson should often decay into an obvious pair of W bosons. Below that it had a bewildering variety of pathways by which to break up. And at masses of less than 135 GeV, it should disintegrate preferentially into pairs of bottom quarks, the next-heaviest link in the great elementary chain of being, as well as gluons and tau leptons. But what these kinds of particles themselves transform into looks much like all the other debris clobbering the detectors. And when an individual W or tau decay involves an easy-to-identify electron or muon, it must also produce a neutrino that exits the detector leaving no track or energy deposit, which makes it more difficult to establish the mass and hence identity of the parent particle. Even if a Higgs boson actually existed at 115 GeV, as the ALEPH experiment had seemed to suggest, it was going to be a long, hard slog to hunt it down amidst such a mess. Finding the Higgs boson at Fermilab was not going to be a piece of cake.
While the Tevatron had plenty of time, it attained insufficient luminosity, especially during the first half a decade. Due to construction and commissioning delays, the LHC experiments did not in fact begin logging data until 2010; the big CDF and D0 experiments at the Tevatron therefore had almost the entire decade all to themselves. A brief flurry of excitement erupted in early 2007 when a group of CDF researchers reportedly observed a small surfeit of events around 160 GeV, disintegrating into tau leptons rather than W bosons. Some interpreted the bump in the graphs of data as evidence of a Higgs boson as predicted by supersymmetric theories, which would decay preferentially in this manner. But interest faded a month later, after the D0 experiment could find nothing similar. And as the data continued trickling in at CDF that year, the intriguing excess withered away – as had so many others.
When the two experiments reported combined results in September 2011, just before the aging, 26-year-old Tevatron was to be shut down forever, there were only small, barely 1σ excesses of Higgs-like events at masses between 115 GeV and 155 GeV – not nearly enough to claim anything significant. Above that level they found too few events decaying via W pairs, so Fermilab could at least take satisfaction in ruling out any Higgs bosons between 156 GeV and 177 GeV. But that was little to show for 10 years of difficult, frustrating research. And it was not enough to convince Fermilab director Pier Oddone to grant the Tevatron a brief stay of execution. As CDF researcher John Conway of the University of California, Davis, lamented, “We’ve gone a very long time with no truly new discovery in particle physics, no observation that truly changes the paradigm.”
Ups and downs at CERN
Fermilab had enjoyed extra time all to itself in the Higgs search due to teething problems at the LHC, the construction of which was delayed by two years because of cost overruns and schedule stretch-outs. In September 2001 CERN director-general Luciano Maiani dropped a bombshell in its council meeting, announcing that the LHC costs were going to grow by almost 20% to CHF 3.34bn, including detector costs. And another CHF 120m would be needed for computing infrastructure. Further cost overruns and delays came later in the decade as a result of problems with the superconducting magnet systems, which had to be cooled with truckloads of liquid helium to just 1.9 K, or –271 ° . When all the expenses were totalled up in 2006, including materials and labour, the final LHC price tag came to about CHF 6bn, more than double its initial advertised cost. Contributions from Canada, China, India, Japan, Russia and the US – the Americans alone having supplied more than half a billion dollars’ worth of equipment – helped ease these financial growing pains. But everything finally seemed to be coming together. On 10 September 2008, with the whole world listening and watching via the BBC, CERN accelerator physicists successfully circulated twin 450 GeV proton beams in both directions through the huge machine without incident. Corks popped. Champagne flowed.
But nine days later, LHC project manager Lyn Evans took a panicky phone call from the control room telling him to come quickly. When he arrived, flashing alarms warned that many magnets had failed and helium gas was filling the tunnel. Later analyses indicated that an electrical splice between two of its 1232 dipole magnets had warmed and “gone normal”, losing superconducting properties. After it melted, an intense spark surged through the magnet vessel, puncturing it and releasing tonnes of helium. When workers later entered the tunnel, they found dozens of magnets damaged, some ripped from their mounts. Soot covered the carnage. Evans called the disaster “a real kick in the teeth for everyone”.
It took more than a year and over CHF 100m to get the LHC back on its feet, and then it was only hobbling. Many splices were found to have additional flaws and would have to be replaced, as did several superconducting dipole magnets. It would be impossible to run the LHC at its design energy of 14 TeV until all the defective splices could be fixed, but that would only happen a few years later. Physicists began to lament that perhaps – after this disaster and the death of the Superconducting Super Collider – God did not want her particle to be discovered, after all.
Cue the collisions
The LHC finally collided protons at 900 GeV on 23 November 2009, with two detectors – ATLAS and CMS – recording the initial events. Four months later, operators gingerly ramped beam energies up to 3.5 TeV, achieving collisions at 7 TeV – but at low intensity. Chastened by the 2008 disaster, researchers became resigned to logging data at only half the original LHC design energy. “It was time to do some physics,” reflected the new director-general Rolf-Dieter Heuer, and making the needed repairs all at once would delay the start of research for at least another year. Wary of a second disaster, CERN accelerator physicists and engineers led by Steve Myers concentrated on improving the collider’s reliability and preparing it for the 2011 run.
The immense, cathedral-sized 7000 tonne ATLAS detector and 12,500 tonne CMS detector had been thoroughly checked out and were ready to begin taking data in their vast underground caverns. It had been a long wait for both 3000-member collaborations – especially the PhD students and postdoctoral researchers, for many of whom this was to be their first experience of a real live particle-physics experiment. These two general-purpose detectors were optimized to be especially sensitive to weighty particles such as a Higgs boson and others expected to occur in supersymmetric theories. They both have excellent energy and momentum resolution (about 1–2%) for electrons, muons and photons. Although a Higgs boson was expected to decay predominantly into pairs of bottom quarks or W bosons, most of which would affect the detectors as jets of hadrons, these decay modes would likely be buried under suffocating backgrounds billions of times larger – and thus nearly impossible to dig out. By concentrating on rare decays in which these leptons or photons appeared, researchers had much better hopes of discerning a signal.
Serious data-taking began in 2011, as operators nudged the luminosity steadily upwards, and proton collisions began rolling in. There were the usual false alarms and overreactions of bloggers, reporters and other scoop chasers who were hovering over the action the entire year. Sau Lan Wu’s group at the University of Wisconsin, for example, circulated an excited internal report in late April of a potential 115 GeV Higgs boson (reminiscent of the final 2000 ALEPH events) decaying into a duo of high-energy photons; it quickly found its way into the blogosphere and went viral, forcing researchers to cancel their Easter vacations to check it out. This “Easter bump”, recalled then CMS spokesperson Guido Tonelli, helped focus his colleagues’ attention on this rare but (soon-to-be) important decay channel. However, the effect proved to be a random fluctuation that withered away in early May after more data came in.
Rumours flew that candidate Higgs boson events might be revealed at the summer 2011 physics conferences. The ATLAS and CMS experiments showed modest excesses of events between 115 and 145 GeV but little else worthy of a press release. CERN could rule out Higgs masses down to 145 GeV and beyond 177 GeV, improving significantly upon the existing Fermilab limits. The Higgs boson was running out of places to hide. If it indeed existed, it now had to be confined to a narrowing range of possible masses between 114 GeV and 145 GeV.
Data deluge
That autumn LHC operators steadily prodded its luminosity to new heights. By the end of the 2011 runs, both experiments had accumulated almost half of what the Fermilab experiments had managed over the previous decade – and at 3.5 times the energy. Events flooded in almost too fast to be recorded. And a small fraction of them looked just as expected for a Higgs boson with a mass close to 125 GeV. In a proton collider such as the LHC, the dominant production mechanism for such a particle is “gluon fusion”, in which gluons in two colliding protons merge to create a Higgs boson, which should quickly break up mainly into two bottom quarks, the most massive final state readily accessible. But that signal would be buried under a huge, burdensome mountain of noise; it is thus very difficult to extract, due to the detectors’ poor energy resolution for hadron jets.
ATLAS and CMS Higgs hunters therefore focused instead on final states with two photons or four charged leptons (electrons or muons), for which they did have good energy resolution and could observe all of the decay products. Although these are rare decay modes, occurring much less than 1% of the time, the narrow peaks that result from plotting such events versus their total energy (or, more accurately, invariant mass) should jut up above the smooth continua of two-photon and four-lepton background events. And that indeed seemed to be the case – especially for the two-photon final state, for which both experiments witnessed more than 70 excess events near 125 GeV. In addition, ATLAS physicists unearthed a few extra four-lepton events at the same energy, while CMS found a similar surfeit near 120 GeV. Something new and Higgs-like was happening in this vicinity.
CERN went cautiously public with these preliminary results in a dual seminar on 13 December 2011, viewed on webcast by thousands of particle physicists and science reporters around the globe. Expectations were high, as rumours of a discovery had been percolating for over a week. But nobody (except some in the press) claimed a discovery. “We have restricted the most likely mass region for the Higgs boson to 116–130 GeV, and over the last few weeks we have started to see an intriguing excess around 125 GeV,” noted ATLAS spokesperson Fabiola Gianotti. Tonelli agreed with her assessment, admitting that CMS physicists “cannot exclude the presence of the Standard Model Higgs between 115 and 127 GeV because of a modest excess of events in this mass region”.
What was new and strikingly different this time was how ATLAS and CMS corroborated one another, revealing intriguing peaks beginning to emerge near 125 GeV. In addition, the CMS experiment had found Higgs-like events in a few other decay modes at about the expected rates. When the combined analyses of these two experiments were published the following February, both the ATLAS and CMS experiments claimed to be observing better than 3σ effects near 125 GeV. But after further data analysis, the statistical significance fell below that level in results presented at the March 2012 Moriond Conference in La Thuile, Italy, considered the principal winter gathering for particle physics. And when the “look-elsewhere effect” (see Physics World August p26) was included, the significance dropped to just above 2σ. These were not yet robust results.
Down but not out, CDF and D0 physicists – many of whom also worked in the ATLAS and CMS experiments – were preparing their comeback. Using sophisticated techniques, they reanalysed both of their full data sets, struggling to wring out every possible Higgs boson event. The Tevatron should have been particularly effective at generating “associated production” events in which a quark within a proton merges with an antiquark from a colliding antiproton to yield a W or Z particle plus a Higgs boson. CDF and D0 physicists sought events in which the W or Z decayed into an easily identified lepton pair and the Higgs boson into two bottom-quark jets; in such events they had much better chances of discerning a Higgs-like signal from noise. And indeed, they succeeded.
At the Moriond conference, both Tevatron experiments revealed new data with broad bumps between 110 GeV and 140 GeV. Combined into a single result, the CDF and D0 data had a bulging 2.2σ excess between 115 GeV and 135 GeV (figure 1), much as expected for a 125 GeV Higgs boson disintegrating into bottom quarks; but the events were spread out over a much wider mass range as a result of the detectors’ poor energy resolution for hadron jets. These Fermilab data clearly reinforced the 2011 CERN results.
Endgame in sight
It looked like the elusive Higgs boson had finally been cornered. While none of the individual results was convincing by itself, four separate experiments had witnessed intriguing excesses in the vicinity of 125 GeV. And the decays of whatever it might turn out to be accorded pretty well with the Standard Model prescriptions for a Higgs boson (but at only moderate significance). Moreover, the indirect limits on such a particle based on precision measurements had long suggested that a lower-mass Higgs boson should in fact exist.
Some theorists were already convinced it had indeed been discovered. “I’m willing to bet a year’s salary!” exclaimed Nima Arkani-Hamed of the Institute for Advanced Study in Princeton, New Jersey, in a panel discussion in March. Gordon Kane, a co-author of the 1990s classic book, The Higgs Hunter’s Guide, thought so, too. “When you have four independent signals, they almost never go away,” he argued at a press conference in April, suggesting that one could get a 5σ result just by combining all the data from the four experiments. But Kane had an obvious theoretical axe to hone, too, having co-authored a paper the previous December, only days before the CERN seminar, predicting a 122–129 GeV Higgs boson based on considerations of supersymmetry and string theory.
Cautious experimenters, wary of getting too far ahead of the data, however suggested everybody take a deep breath and sit tight until after this year’s LHC runs, which began in April with protons colliding at 8 TeV and machine operators aiming to double the luminosity. By the end of 2012, predicted CERN officials, ATLAS and CMS physicists should have the final answer – and be able to put an end to Peter Higgs’ long, long wait.
And his wait turned out to be shorter, as the official discovery of a Higgs-like particle near 125 GeV was announced on 4 July (see “CERN discovers Higgs-like boson”). The decades-long search for a Higgs boson was finally over.