Flash Physics is our daily pick of the latest need-to-know developments from the global physics community selected by Physics World‘s team of editors and reporters
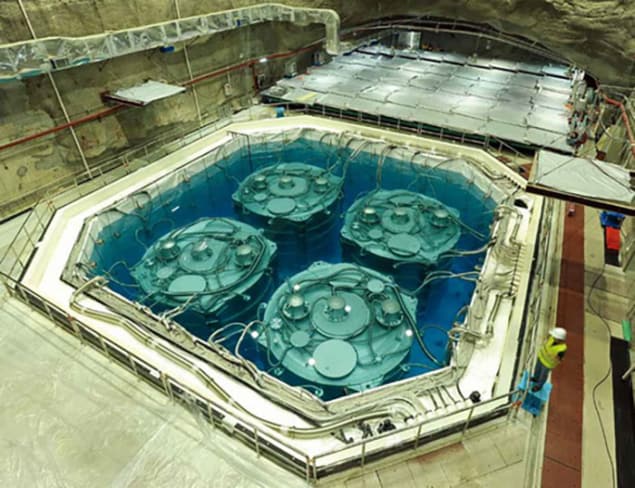
Antineutrino anomaly is a calculation error, not sterile neutrinos
An error in how antineutrino production is calculated could be responsible for the mismatch between measurements of the numbers of antineutrinos produced in nuclear reactors and theoretical predictions. That is the conclusion of an international team of physicists working on the Daya Bay Neutrino Experiment at a nuclear-power complex in China. Nuclear fission in commercial reactors creates huge numbers of antineutrinos, which can then be detected by the Daya Bay experiment and other detectors located near to reactors worldwide. Since 2011, physicists have noticed that significantly fewer antineutrinos are detected by these experiments than predicted by theory. Some have speculated that the missing particles had morphed into sterile neutrinos on the short journey from reactor core to detector. Sterile neutrinos are hypothetical particles that could account for some of the mysterious dark matter that is thought to pervade the universe – and therefore any evidence of sterile neutrinos is of great interest to physicists. The Daya Bay team has looked at the antineutrino flux from two main fission isotopes in the reactor core – uranium-235 and plutonium-239. The researchers were able to show that the measured flux from plutonium-239 matches theoretical predictions – which suggests that antineutrinos from this isotope are not morphing into sterile neutrinos. As a result, they conclude that the current theory incorrectly predicts an over-production of antineutrinos by uranium-235 fission of about 8%. Writing in a preprint on the arXiv server, they point out that their conclusion could be tested by future experiments based at reactors fuelled with high-enriched uranium.
Proton beam boosted by combining laser bursts
Proton beams have been produced by using a prolonged laser burst of lower than expected energy. Proton-beam systems are receiving increasing attention because of their application in cancer treatment. One method for producing the beam of charged particles is laser-plasma acceleration. This is when powerful lasers are fired at ultra-thin metal foils, producing a plasma in which electrons separate from ions. The resulting huge electric fields can accelerate protons, ions and electrons to high energies. Typically this is done with a burst of high-contrast laser light, a single picosecond in length. While polarized light and repeated pulses have shown promise in improving the quality of proton beams, little is known about using longer bursts of light because such intensely powerful lasers can only be generated for a short time. Now, scientists at Osaka University have used one of the world’s most powerful lasers, the Laser for Fast Ignition Experiments (LFEX), to study longer bursts. “By carefully timing the firing of four beams, it was possible for us to effectively fire each in sequence to generate longer pulses that otherwise had the same sharp features as single pulses,” says group leader Hiroshi Azechi . The configuration meant that the laser light could be 100 times less intense than previously thought necessary to produce high-energy protons. “Using multiple pulses to create a longer pulse heats up the electron plasma significantly, which is likely what causes the charged particles to achieve a higher energy at a lower laser intensity,” explains team member Akifumi Yogo. The finding, presented in Scientific Reports, could lead to more efficient proton beams and provide increased precision for medical applications. For more on proton therapy for cancer treatment, see the free Physics World Discovery ebook Proton Beam Therapy.
Silicon-III is a semiconductor, not a metal

Silicon normally adopts a diamond-like crystal structure, but under the right conditions it can assume several other structures including silicon-III, which has a cubic structure with 16 atoms in a unit cell. Previous studies had suggested that silicon-III is a poorly conducting metal without an electronic band gap. But now physicists in the US and France, led by Tim Strobel at the Carnegie Institution for Science in Washington DC, have made and studied pure bulk samples of silicon-III and shown that the material is actually a semiconductor with a very narrow band gap. They made their samples by applying extreme pressure to normal silicon and confirmed that they were pure silicon-III using X-ray diffraction, Raman spectroscopy and nuclear magnetic resonance spectroscopy. They then did a series of experiments on the samples that looked at the optical, electrical and thermal properties of the material. Together, these measurements show that silicon-III has a band gap of about 30 meV, which is much smaller than the 1.1 eV band gap of conventional silicon. Unlike conventional silicon, silicon-III has a direct band gap. This means that electronic transitions in the material can involve the direct emission of a photon. The band-gap energy of 30 meV corresponds to an infrared photon, so silicon-III could be particularly useful in future plasmonic devices that could operate at that energy. The work is described in Physical Review Letters.
- You can find all our daily Flash Physics posts in the website’s news section, as well as on Twitter and Facebook using #FlashPhysics.