What effect does technology have on the performance of athletes in sports such as the 100-metre sprint, the pole vault and the javelin? Ultimately, it seems that there is a conflict between the laws of physics and the laws of sport, says Steve Haake
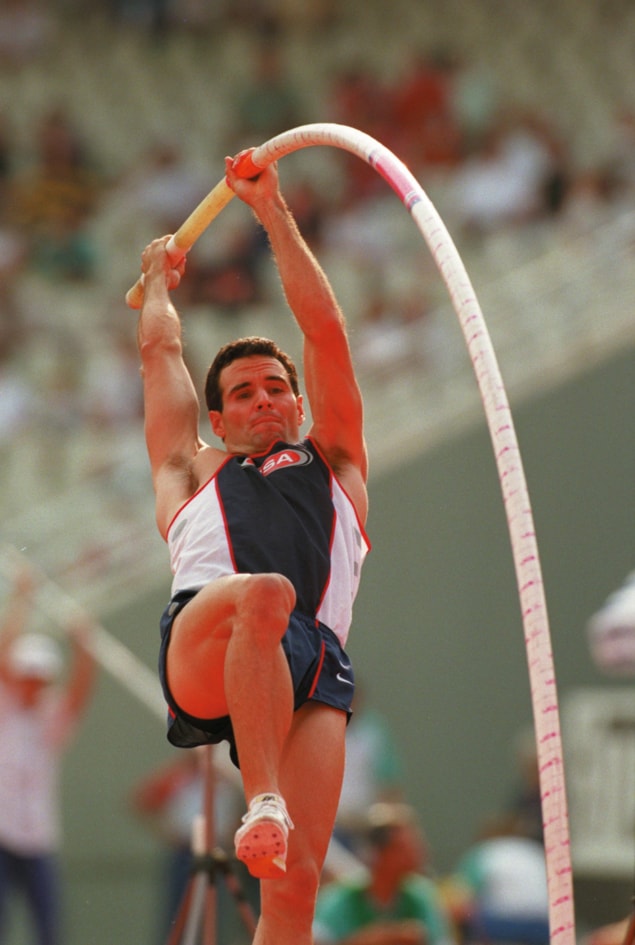
from advances in technology. (Courtesy: Tony Marshall/EMPICS)
The modern Olympic games were founded by Baron Pierre de Coubertin in 1896, with the intention of improving health and education, promoting world peace, and encouraging fair and equal competition. However, the motto of the modern Olympic games – citius, altius, fortius (swifter, higher, stronger) – shows that it is not only the taking part that counts. Winning is just as important now as it was 2500 years ago at the original games in ancient Greece. Then, as now, winning athletes were treated like heroes, given seats for life at theatres and public gatherings, and awarded cash bonuses, gifts and prizes. It is no wonder, then, that athletes – both ancient and modern – have used any means at their disposal to improve the speeds at which they can run, the distances they can throw and the heights they can jump.
In sports such as the discus, hammer or javelin – where pieces of equipment are used – athletic performance is governed by the laws of physics, but ultimately limited by the arbitrary rules of sport. And herein lies the conflict. It is human nature for athletes to improve themselves so that they are swifter, higher and stronger – and to use technology if it helps. It is the role of sports’ governing bodies to allow continual improvements to equipment, but to stop those developments that give any competitor too much of an unfair advantage. Conflict can arise as sport is not meant to be a test of who has the best equipment – it should be an even match between one athlete and another, and from one year to the next.
The fact that the rules of sport have been altered and manipulated over the last century to allow (or disallow) developments in equipment implies that technology certainly does influence sport. But what evidence is there that it does? This article looks at the physics of three Olympic athletics events – the 100-metre sprint, the pole vault and the javelin (citius, altius, fortius) – to see if the fine balance between technology and tradition has been maintained.
The 100-metre sprint
The Greeks actually had a sprint of about 190 metres called the stadion in the ancient Olympics, which was a sprint down a straight track and back again. The technology of the day consisted of nothing more than a wooden post at one end to help the runner on his return back up the track. Races originally began with the athletes standing upright, with their toes resting in grooves in a stone starting sill – hence the expression “toe the line”. False starts were punished by flogging from a judge standing behind the athletes. Later it seems that a starting gate (called the husplex) was used, much like that used in horse-racing today.
In the modern Olympics, sprinters start from a crouching position, pushing against starting blocks to help them accelerate. Blocks were introduced in the late 1920s and were first used at the 1948 Olympic games in London. Instrumented starting blocks appeared in the early 1980s, and consisted of a spring plate and a microswitch. In the late 1980s units based on strain gauges emerged, although they were very sensitive to the push of the athlete against them and caused many wrong false starts in competitive races. An improved strain-gauge version that worked quite well was introduced in about 1993, and two years later an “intelligent” version was developed. It has a small module with a microcontroller built into the starting block and uses complex algorithms to eliminate false triggers. Starts are considered false if the athlete starts within 0.1 s of the firing of the starting gun – although the guilty athletes are no longer flogged!
The difference between winning and losing in sprinting is now so small that modern athletes obviously have to be timed as accurately as possible. Today’s timing systems usually consist of a clock, which is triggered by the starter’s gun, a light source, and an optical pick-up device that stops the timer when the winning athlete cuts the light beam. The light source is generally “modulated” – switched on and off – at frequencies of about 1000 Hz so that it is not fooled by changes in background-light intensity.
Tom Westenburg, who works as a principal engineer at the US Olympic Committee in Colorado Springs, helps the committee to develop electronics technology to aid elite American athletes. He has investigated the technological limitations of timing systems in the 100-metres sprint. For example, the timing device, which is typically a quartz oscillator, has to be stable to about 100 parts per million per degree kelvin to stop it losing accuracy as the temperature fluctuates. Westenburg has also shown that a modulation frequency of at least 4000 Hz must be used to ensure that the timing device is accurate to within one-thousandth of a second – as required for the 100-metre sprint. Fortunately such accuracies are becoming easier to realize than in previous decades thanks to improvements in microchip technology.
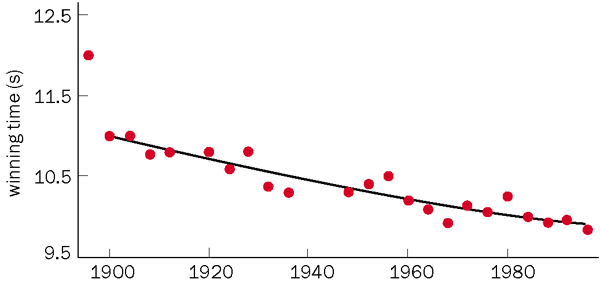
As far as the sprinters themselves are concerned, the technology available to them is fairly limited. Most developments have focused on improving the surface of the track and designing running shoes that are lighter and give a better fit. The winning times for the 100-metre sprint at the modern Olympics show a downward trend that appears to be levelling out (figure 1). Improvements are now about 0.006 seconds per year compared with 0.015 seconds per year a century ago. Given the scatter in the data it is difficult to see any particular moment when there has been a significant increase in performance. It is likely, therefore, that the 100-metre sprint is dominated by human ability and that improved performance is most likely caused by improvements in diet, coaching, fitness and physiology, with technology playing a relatively minor role.
Amazingly, Donovan Bailey, who won the 100-metres gold medal at the 1996 Olympics in Atlanta, would have beaten the first gold-medal winner of 1896 by some 20 metres!
The physics of pole-vaulting
Pole-vaulting was not one of the original Olympic sports in ancient Greece. Some think that the sport was derived from the Dutch habit of dyke-jumping, although one of the earliest pole-vaulting stands was built in Germany in 1791. The objective is, obviously, to get the athlete’s centre of mass over the highest bar possible. However, today’s pole-vaulters use a quite different technique to that used 100 years ago, when athletes went over the bar with their feet pointing downwards. Athletes now do a complex gymnastic manoeuvre, turning upside down as the jump takes place (figure 2a). We shall see that this is a direct result of the technology used.
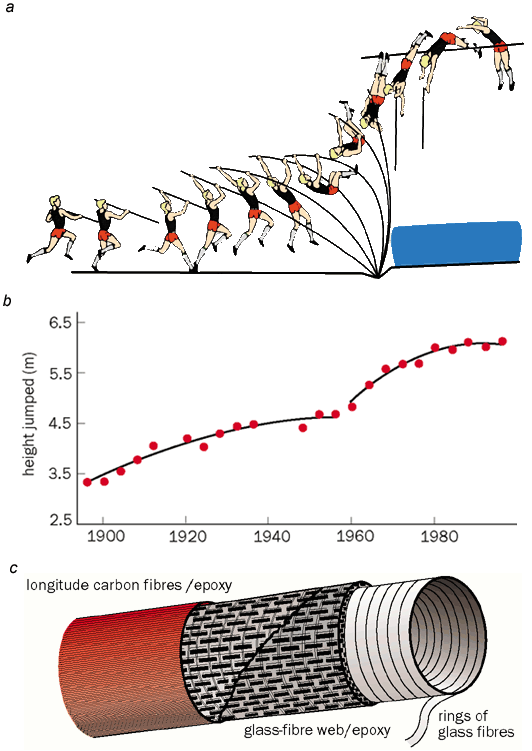
The rules for pole-vaulting that are set by the International Amateur Athletic Federation (IAAF) – the sport’s governing body – are extremely liberal. There is no restriction on the length of the pole, the materials from which it is constructed or its energy-storage capacity. The only stipulation is that poles should be generally smooth and not be covered with too much adhesive tape.
Poles were originally made out of solid wood, probably hickory. Slightly more flexible bamboo poles were introduced in the early 1900s, mostly by American vaulters, who dominated the sport at the time. Basic mechanics tells us that the highest stresses occur on the outside of a bent beam, and that a symmetrically bent object, such as a pole, actually has a zone down the middle (known as the neutral axis) where the stresses are very low and even zero. There is therefore no need for the pole to have any mass down the centre. Bamboo, which is a naturally hollow material, is much lighter per unit length than a solid pole – yet provides the same maximum stress. This enables an athlete carrying a bamboo pole to either take a faster run-up or to use a slightly longer pole.
The use of bamboo poles led to a steady increase in the winning height of the Olympic pole-vaulting competition (figure 2b). However, the improvements were starting to level off by the mid-1950s, and in the early 1960s bamboo began to be replaced by glass-fibre poles. This led to a dramatic increase in the winning heights. Glass-fibre poles consist of long filaments of glass fibre – ranging from 3-20 µm in diameter – embedded in a matrix of less stiff polymer resin. The material can readily be fabricated into different shapes and has a high stiffness-to-weight ratio.
Essentially pole-vaulting involves the conversion of the kinetic energy of the running athlete to the potential energy of the jump using strain energy stored in the pole (the energy stored in elastic deformation). Consider an athlete of mass m = 80 kg running at a speed v = 10 m s-1, who has kinetic energy of 1/2mv2 = 4000 J. If this energy is converted with 100% efficiency into potential energy mgh, where g is the acceleration due to gravity and h is the height jumped, then the athlete can climb a height of 4000/mg, or just over 5 m. However, in reality, most pole-vaulters can jump heights of nearly 6 m. So where does the extra energy required to propel the athlete to these greater heights come from?
It turns out that the extra energy comes from the athleticism of the vaulter bending the pole. Energy is stored in the pole as it is bent or strained by the athlete’s muscles, and returned to the vaulter as the pole recoils. The strain energy comes from the work done by the muscles of the athlete as he or she takes off, carrying out work on the pole as it is bent. The maximum strain energy of the pole is ms2/2rE, where s is the maximum or “failure” stress on the outside of the pole, r is its density and E is its Young’s modulus (i.e. its “stiffness”).
Bamboo has a relatively low Young’s modulus and density, and a moderate failure stress. Glass fibre also has a low Young’s modulus and density – but a much higher failure stress than bamboo. In fact, the maximum strain energy that can be stored in a glass-fibre pole before it breaks is about 2500 J, compared with just 100 J for bamboo. One consequence of this is that glass-fibre poles can be bent through much larger angles before breaking, which is why athletes can use them to jump gymnastically over the bar.
If we assume that the efficiency of a glass-fibre pole is 50%, an extra 2500 J of stored energy would be enough to get the athlete’s centre of mass, feet first, over the bar. In other words, our athlete would have a kinetic energy of 4000 J plus a strain energy from the pole of 1250 J, giving a total energy of 5250 J. If all this is converted into potential energy, the athlete would climb a height of 5250/80g ~ 6.5 metres.
The advances in pole-vaulting performance are certainly not as good now as they were in the 1960s. That has not, however, stopped researchers from searching for further improvements, which have included introducing carbon fibres to make the pole still stronger and lighter (figure 2c), and allowing the pole to vary in thickness along its length. The latter innovation stemmed from work done in 1996 by Stuart Burgess, then at the Department of Engineering at Cambridge University. He demonstrated theoretically and experimentally that, during a jump, the greatest bending moments – and hence the greatest stresses – are at the middle of a pole, while the lowest stresses are at the ends. In other words, the ends of a pole can be made narrower – and hence lighter – without compromising the pole’s performance. Burgess therefore optimized the thickness of a pole so that it tapered towards its end, giving a mass saving. Also, the bending moment – and hence the failure stress – increases with the stiffness of the pole for a given section of the pole and radius of bend. The ideal pole therefore has a low stiffness, a low mass and a high failure stress.
Clearly, pole-vaulting is an example of a sport in which technology has been used to improve athletic performance. As the Olympic winning heights in the discipline level off, it will be interesting to see if our ingenuity can provide another technological leap to allow pole-vaulters to jump even higher.
The javelin
The javelin was an event first enjoyed by the Mycenaeans at least 3000 years ago. The Greeks of 500 BC used thin wooden javelins with a cord wrapped around the centre of mass. When the javelin was thrown, the athlete would hold onto the end of the cord to make the javelin rotate freely through the air – in much the same way that a toy gyroscope is made to rotate by pulling a string. The rotation stabilized the javelin by averaging any asymmetries in its construction about a central axis.

Compared with the pole vault, the modern javelin has relatively strict rules governing its design (for example, the length of a javelin for the men’s event must be between 2.60-2.70 m and have a minimum mass of 800 g). It must also be smooth, and there are strict geometric rules stipulating where its centre of mass must lie. The reason for this can be seen by examining the winning throws at Olympic games from 1904 onwards (figure 3). At the London games in 1908 the winning throw was just over 50 m. By 1976 this distance had increased to almost 95 m and in 1984 Uwe Hohn (from the former East Germany) threw a staggering 104.80 m in a non-Olympic event. Athletes at this time were able to throw javelins almost the full length of a sports stadium, putting the lives of spectators at risk! The IAAF decided fairly quickly that the javelin had to be re-designed to underperform. This was done by moving the centre of mass forward by 4 cm – the effect of which is explained below.
At about the time of the IAAF rule change in the mid-1980s, Mont Hubbard of the University of California at Davis carried out a series of tests and simulations to look into the physics of the flight of the javelin. He found that javelins are launched at about 30o to the horizontal with an “angle of attack” of about 7o – in other words, the javelin is tilted 7o more steeply than the direction traced by its centre of mass. The angle of attack influences both the javelin’s “lift”, which acts perpendicularly to its direction of motion, and the drag, which acts parallel to it (figure 4a). These forces do not act at the centre of gravity but are displaced from it through a “centre of pressure” – the point through which the aerodynamic forces (lift and drag) act.
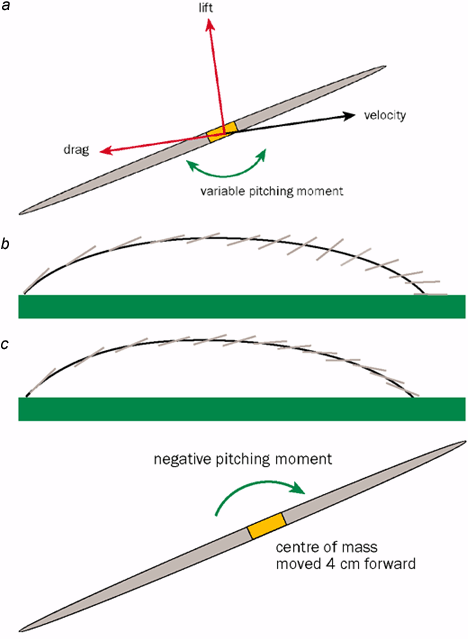
If the centre of pressure is behind the centre of mass, it causes a “pitching moment” that makes the tip dip down. However, if the centre of pressure is ahead of the centre of mass, it makes the angle of attack increase, causing the javelin to stall. Although the lift and drag forces were relatively constant from one “old-rules” (pre-1984) javelin to another, the pitching moment was not since it moved forward and back about the centre of mass throughout its flight. The result was that the furthest distance a javelin could be thrown depended on how it was designed. A “positive” pitching moment occurred in the early stage of the flight of an “old-rules” javelin, rotating the javelin about its centre of mass so that the angle of attack increased (figure 4b). In the later stages of its flight the pitching moment acted in the opposite sense – a “negative” pitching moment – rotating the javelin so that the angle of attack decreased until the tip only just hit the ground first.
When the rules were changed so that the centre of mass was moved 4 cm forward, it effectively removed any positive pitching moments so that the angle of attack did not increase early on in the flight. This caused the overall lift to drop. A “new-rules” javelin keeps its nose down relative to its velocity vector, and there are no high lift forces at the end of the trajectory. The net effect is to reduce the total distance a javelin can travel. Indeed, the winning javelin throws at the Olympics are now some 15 m less than they were before the rule change.
As far as the rule-makers are concerned, the new javelin has two advantages: it does not fly as far and it lands tip first, which is obviously safer. The new rule also gives the athlete an advantage because the “old-rules” javelin was very sensitive to the initial throw conditions, and even a small change could reduce distances by as much as 20 m. The “new-rules” javelin, in contrast, is much less sensitive to initial conditions, partly because it always has a negative pitching moment. Athletes can now produce much more consistent throws. But as figure 3 makes clear, it might not be too long until a further rule change is required!
The balance between physics and sport
The examples in this article highlight the varying impact that technology has had on sport. In the 100-metre sprint, it seems that the strength and power of the athlete dominates, and that no technological development has arrived that requires a change of rules. Performances in the pole vault, in contrast, improved dramatically with the introduction of flexible poles in the 1960s. However, it was the ability of the athlete to adapt to the new equipment – rather than the physics of the equipment itself – that produced the gains. And since the technology has been widely available to all athletes, the ruling bodies have – so far – not deemed it necessary to change the rules to deliberately keep heights low. Meanwhile, the authorities altered the rules of javelin by exploiting the laws of physics to reduce throw lengths and make the sport safer for both athletes and spectators.
There is, it seems, a balance between technology and tradition. The ruling bodies either allow technology to advance a sport (such as in the pole vault) or use it to under-engineer a sport (such as the javelin). Is it cheating? Well, as long as the same technology is available to all competitors at the same time, then ultimately it comes down to the ability and the skill of the athlete. Problems only arise when technology is available exclusively to only one group of athletes.
Happily, a century on from Baron de Coubertin’s original vision of the Olympics, the motto swifter, higher, stronger ultimately still depends on the skill of the athlete.