The ability to tune the interactions between atoms in a Bose-Einstein condensate has led to atomic wave packets that can propagate without dispersion and other novel quantum states.
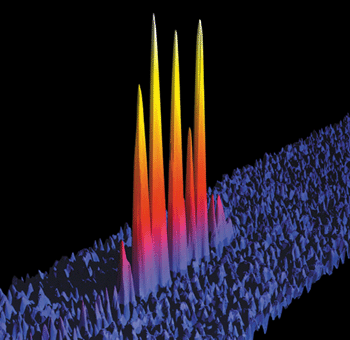
One of the remarkable things about low-temperature physics is that it offers a place where the hidden world of quantum mechanics can be revealed, often giving rise to spectacular effects. In 1995 the Nobel-prize-winning observation of Bose-Einstein condensation showed that a dilute gas of atoms cooled to sufficiently low temperatures could “condense” into an intriguing state in which all the atoms can be described by the same quantum wavefunction.
Bose-Einstein condensation is actually a phase transition – just like the formation of ice in a vessel of water when it is cooled below freezing – but it occurs as a direct result of the underlying rules of quantum mechanics. For decades undergraduates the world over have studied this phenomenon as a textbook problem in statistical physics.
So why is Bose-Einstein condensation in gases still the subject of intense research? If the atoms were truly non-interacting, like the textbook problem usually assumes, interest would likely have died out long ago. Without interactions it would not have been possible to demonstrate superfluidity in these systems, including the recent beautiful observations of large numbers of vortices arranged in well ordered lattices (see Triple first for Bose condensates Physics World May 2001 pp21-22). The atoms do interact, but in such a way as to allow a detailed theoretical understanding from first principles.
The interactions can even be dynamically controlled, allowing one to prescribe whether the atoms interact strongly or weakly and to change the force between the atoms from an attractive interaction to a repulsive one. This developing theme of control is one reason why breakthroughs in the field continue to emerge at a remarkable rate – such as the recent demonstration of bright solitons in a Bose-Einstein condensate.
Out of the shadows
Solitons, and their close relatives solitary waves, occur throughout physics. The term solitary wave refers to a localized disturbance in a continuous medium that can propagate over long distances without any change to its shape or amplitude. Such disturbances were noted as long ago as 1834 by the Scottish scientist John Scott Russell for a shallow canal. He noticed that water waves could propagate for many miles without attenuation or dispersion.
Solitons are essentially the same as solitary waves but they have the additional feature that their amplitude and spatial profile do not change even when multiple solitons collide. Optical solitons are particularly important in fibre-optic communications because the information they carry remains intact as the light pulses travel through the glass fibres.
Now two groups – Randall Hulet’s group at Rice University in the US, and Christophe Salomon and co-workers at the Ecole Normale Supérieure in Paris – have announced the successful generation of bright solitons in Bose-Einstein condensates. This latest work follows several previous experimental efforts to study “dark solitons” in condensates. These objects correspond to regions inside the condensate that ideally contain no atoms, and they occur in systems where the interactions between the atoms are repulsive. Obviously this kind of disturbance can only exist within the confines of a medium. Bright solitons, on the other hand, are completely self-determined condensate bunches that bind together and can propagate through free space. They only occur in systems where the attractive interactions exactly compensate for any dispersion of the wave packet.
Although there are important differences in detail, the two experiments are remarkably similar in spirit. They both used the same isotope of lithium, namely lithium-7, to form the gas. The first step was to create a Bose-Einstein condensate using the now standard techniques of laser cooling and evaporative cooling (see Physics World August 1999 pp37–42, print edition only). Next the atoms were transferred from a trap created by magnetic-field gradients to a simple 3D trap formed by strong laser fields in order to allow the interactions to be controlled.
The Paris group generated a bright soliton by tuning the interactions between the atoms to be slightly attractive (L Khaykovich et al. 2002 Science 296 1290). The researchers observed that most of the condensate atoms were lost, probably due to the fact that an attractive interaction in a gas can lead to the onset of mechanical instability and collapse. However, they also saw a condensate remnant containing some 6000 atoms form a stable bright soliton that could then be studied. Salomon and co-workers guided the atoms in one dimension for a distance of approximately 1 mm and established that there was no discernible spreading of the wave packet and no decay of the amplitude – a clear signature for a soliton. And when they repeated the experiment without interactions between the atoms, they saw obvious evidence for spreading of the wave packet.
The Rice group used a different configuration of laser trap that also restricted the atoms to move in one dimension. In this case, the researchers showed that multiple bright solitons could be formed, each containing in the region of 6000 condensate atoms (K Strecker et al. 2002 Nature 417 150). These multiple-soliton trains were previously known to be possible solutions of the underlying equations that describe Bose-Einstein condensates, but their production is particularly interesting since it allows collisions that are essential to the nature of solitons to be investigated (see figure). Hulet and co-workers observed that the solitons repelled each other, thereby providing information about the phase structure of the underlying condensate wavefunction.
Excitement over excitons
Although quite unrelated, another recent development in Bose-Einstein condensation should be followed with interest. Leonid Butov of the Lawrence Berkeley National Laboratory and co-workers in the US and the UK have recently created regions in a semiconductor that allow the confinement of a high density of composite particles called excitons (L Butov et al. 2002 Nature 417 47). This advance is important because it is a precursory step on the way to making a Bose-Einstein condensate from excitons, rather than atoms.
Excitons are created in semiconductors when a laser field promotes an electron from the valance band to the conduction band. The resulting electron in the conduction band and the hole that it leaves behind can form a bound composite object due to the Coulomb attraction. This composite object is the exciton and it can, in principle, undergo Bose-Einstein condensation if the density and temperature are right. Although significant efforts over many years have gone into demonstrating Bose-Einstein condensation of excitons, the results so far have been controversial.
Butov’s group was able to demonstrate trapping of excitons in all three dimensions thanks to the development of a semiconductor structure that overcame some of the previous limitations. Sandwiched in the middle of the semiconductor are two layers of quantum wells in which the electrons and holes are created and confined to a 2D world. When an external electric field is applied, an electron can be confined to one of the quantum wells while a hole is trapped in the other, thereby leading to a significant increase in the exciton stability.
Of course, a trap needs to provide confinement in all three dimensions. This was possible because the natural disorder in the system led to random fluctuations in the potential energy. Potential wells a few microns across form at certain places in the material and can confine cold dense clouds of excitons. Although Butov and co-workers have not yet demonstrated Bose-Einstein condensation in this novel system, significant progress has clearly been made.
Breakthroughs galore
These are just two examples of the many exciting developments that are currently going on in this field. In addition, Carl Wieman and colleagues at the JILA laboratory in Colorado have found evidence for a molecular Bose-Einstein condensate in a sample of rubidium-85 molecules (E Donley et al. 2002 Nature 417 529; S Kokkelmans et al. 2002 arXiv.org/abs/cond-mat/0204504). Rather than cool the molecules, the JILA team converted part of an atomic condensate into molecules by tuning the interactions between the atoms with pulses of magnetic field.
Meanwhile, Wolfgang Ketterle’s group at the Massachusetts Institute of Technology has shown that a Bose-Einstein condensate of sodium atoms in an optical trap can be replenished with atoms from a second identical condensate using optical tweezers (A Chikkatur et al. 2002 Science 296 2193). This advance is an important step towards creating a new type of laser that continually emits atoms rather than photons (see Atom lasers Physics World August 1999 pp31-35).
Another goal in condensate research is to observe the transition of a dilute fermion gas to a superfluid phase at relatively high temperatures (see A Fermi gas of atoms Physics World April pp27-31). Such a transition would complement studies of superconductivity in exotic condensed matter, and could shed light on the crossover between normal and high-temperature superconductivity.
The future for Bose-Einstein condensation is very bright, and if the breakthroughs continue at the current rapid pace, we may not have to wait too long to see what advances it will bring.