The recent discovery of elements 113 and 115 will tell us more about the structure of the nucleus and the possible existence of the “island of stability”.
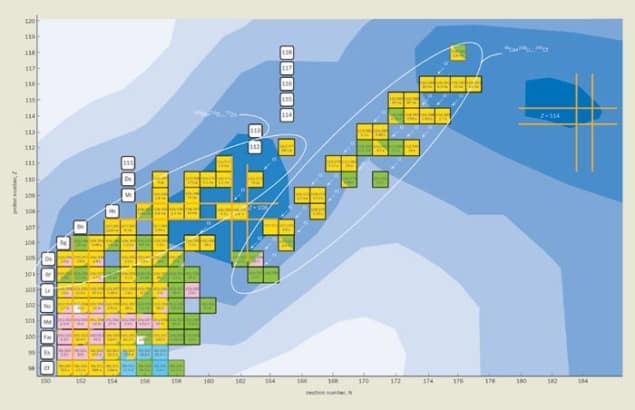
The periodic table of the elements is slowly getting bigger. At one time it contained only 83 naturally occurring elements, starting with hydrogen and ending with uranium. These elements have half-lives that are comparable to the age of the Earth, which is about 4.5 billion years old. Since the 1940s, however, physicists have been able to produce unstable elements that decay to lighter elements on timescales that can range from thousands of years to tiny fractions of a second. By last year a total 114 elements were known, and earlier this year the author and co-workers reported the synthesis of two new “superheavy” elements.
There is, however, more to this branch of physics than simply (or not so simply) creating heavier and heavier elements. It is also essential to understand the behaviour of these new elements, many of which do not yet have official names. Superheavy elements allow nuclear physicists to explore concepts such as “magic numbers” and the “island of stability”, which help us understand why some nuclei are more stable than others. They can also be used to test the predictions of different models of the nucleus and, ultimately, they may help us to understand why nature contains only a finite number of elements.
Elementary numbers
As every physics student knows, a nucleus contains roughly similar numbers of protons and neutrons, apart from the hydrogen nucleus, which is simply a proton. The same element can also exist as several different isotopes: for instance, carbon-12 contains six protons and six neutrons, and is stable, whereas carbon-14 contains six protons and eight neutrons, and has a half-life of 5730 years. Various numbers are used to define nuclei: the atomic number, Z, is the number of protons, while the mass number is the sum of the atomic number and the number of neutrons, N.
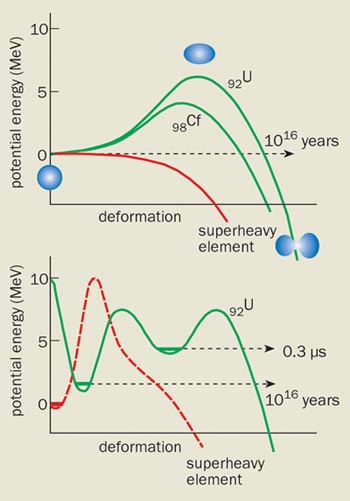
Heavy elements also tend to contain more neutrons: the most stable isotope of lead, for example, contains 82 protons and 126 neutrons. However, if we add one or more neutrons to a stable nucleus, or take neutrons away from it, the nucleus may become unstable and undergo radioactive decay.
Nuclei can decay in different ways: in alpha decay the nucleus emits an alpha particle (i.e. a helium nucleus containing two protons and two neutrons); and in beta decay a neutron can decay into a proton, emitting an electron and an antineutrino in the process. A heavy nucleus can also split into two parts in a reaction called spontaneous fission. This was observed for the first time in 1940 by Georgy Flerov and Konstantin Petrzhak with uranium-238 nuclei.
Nuclear fission prompted Niels Bohr and John Wheeler to propose the liquid-drop model, which treats the nucleus as a drop of charged liquid without any structure. As long as the surface tension in the drop is larger than the repulsive Coulomb force due to the protons, a potential barrier prevents it from splitting (figure 1). However, this fission barrier can be overcome if we supply enough energy to the nucleus or if the nucleus “tunnels” through the barrier.
For the uranium-238 nucleus, which has an atomic number of 92, the fission barrier is about 6 MeV high. This leads to a spontaneous-fission half-life of about 1016 years. However, as the atomic number becomes higher, the potential barrier becomes smaller and eventually disappears, causing the heaviest nuclei to decay in about 10-19 s. According to Bohr and Wheeler, the potential barrier disappears completely when the atomic number reaches about 106.
The lifetimes of the first “transuranium” elements – such as plutonium, curium and californium – were very close to the values predicted by the liquid-drop model. In 1962, however, researchers at the Joint Institute for Nuclear Research (JINR) in Dubna discovered that many isotopes of transuranium elements ? which have a very low excitation energy ? undergo spontaneous fission in just 10-10-10-2 s. This is inconsistent with the liquid-drop model predicts. Furthermore, the model could not account for considerable variations in the spontaneous-fission half-lives of these “isomers”.
Researchers soon realized that the probability of spontaneous fission depends on the internal structure of the nucleus. It was well known, for example, that the total nuclear binding energies measured in experiments deviated from the predictions of the liquid-drop model in a regular way. The binding energies were highest for specific numbers of protons, Z = 2, 8, 20, 28, 50, 82, and neutrons, N = 2, 8, 20, 50, 82, 126. These “magic” numbers of protons and neutrons are called closed shells, and are similar to the electron shells of atomic physics.
By the end of the 1960s these observations led to a new microscopic theory of the nucleus, which showed that closed shells of protons and neutrons allow nuclei to be stable beyond the limits defined by the liquid-drop model (i.e. for atomic numbers greater than 106). The shell effect was predicted to be particularly strong for nuclei with magic numbers Z = 108 and N = 162, and even stronger for nuclei with Z = 114 and N = 184 – which is why these regions came to be known as “islands of stability” (figure 2). Indeed, the lifetimes of superheavy nuclei in the N = 184 region are predicted to be up to 30 orders of magnitude longer than they would be in the absence of shells. The challenge presented to experimental nuclear physicists was clear: they needed to create the superheavy nuclei and measure their properties to put the theoretical predictions to the test.
Synthesis reactions
The first transuranium elements were in the main synthesized in successive neutron-capture reactions at the Lawrence Berkeley Laboratory between 1940 and 1953. These experiments – in which nuclei gain extra neutrons during long exposures in a high-neutron-flux reactor – resulted in the discovery of new elements with atomic numbers up to 100 (fermium). However, heavier nuclei could not be explored with this technique because they decayed before they had time to capture the next neutron.
In the effort to produce elements heavier than fermium, researchers turned to heavy-ion reactions, in which two nuclei – one in a heavy-ion beam, the other in a target – are forced to undergo a fusion reaction to create a heavier, compound nucleus. The trouble with this approach, however, is that the collision between the ions leaves the resulting compound nucleus in a highly excited state, which means that it is more likely to undergo fission immediately. Furthermore, the stabilizing effect of nuclear shells decreases rapidly as the excitation energy increases.
Exploring atomic numbers above 106 only became possible after the discovery of so-called cold-fusion reactions at JINR in 1974. In these reactions, heavy ions such as lead or bismuth are bombarded with projectile ions that have a mass number of more than 40. In these reactions, the kinetic energy of the projectile is absorbed, resulting in a compound nucleus that is much less excited.
In the early 1990s Peter Armbruster, Sigurd Hofmann, Gottfried Münzenberg and co-workers at the GSI laboratory in Darmstadt, Germany, used cold-fusion reactions to synthesize elements 107-112. These data were later confirmed by Kosuke Morita and co-workers at the RIKEN laboratory in Tokyo, who also synthesized elements 110 and 111 in cold-fusion reactions. Last year the International Union of Pure and Applied Chemistry (IUPAC) agreed that element 110 should be known as darmstadtium, and that GSI should also be credited with the discovery of element 111. Both the GSI and RIKEN teams now have plans to synthesize element 113 and above.
But even cold fusion has limitations if we want to fully explore the superheavy-element region. This is because massive nuclei tend to resist fusion – an effect that increases with the electric charge of the projectile ion – which means that the probability of forming new elements falls exponentially as
a function of the atomic number of the compound nucleus. Furthermore, the compound nuclei produced in cold-fusion reactions contain a relatively small number of neutrons. In the case of element 112, for example, the final nucleus has 112 protons and 165 neutrons, which is still 19 neutrons away from the predicted closed shell at the magic neutron number N = 184.
One way to produce nuclei with higher neutron numbers is to use the rare isotope calcium-48 – which has 20 protons and 28 neutrons – as the projectile. In these reactions the compound nucleus has an excitation energy of about 30-40 MeV. Although this suppresses the shell effects, they are still strong enough to allow the final superheavy nucleus to be observed. Furthermore, the large mass difference between the two interacting nuclei decreases the Coulomb repulsion between them at the moment they touch, and thus increases the probability of fusion.
Despite these obvious advantages, all attempts to synthesize new elements using calcium-48 ions between 1977 and 1985 failed. However, improved experimental techniques and the availability of intense beams of calcium ions have increased the sensitivity of these experiments by at least three orders of magnitude. This has allowed us, together with colleagues at the Lawrence Livermore National Laboratory in the US, to probe deep into the superheavy-element region.
If the theory is right, elements on the islands of stability should not decay via spontaneous fission. Instead they should undergo alpha decay. As a result, these elements will leave a clear experimental signature: a daughter nucleus that is lighter than its parent by two protons and two neutrons, followed by a granddaughter nucleus that is lighter by four protons and four neutrons, and so on. The decay products of the parent nucleus therefore get further away from the magic numbers Z = 114 and N = 184 until they pass the boundary of the island of stability, beyond which spontaneous fission starts to dominate the decay process. The synthesized heavy nucleus therefore has to be as close as possible to the neutron shell N = 184 to produce a long alpha-decay chain.
Calcium exists naturally in great abundance, but only about 0.19% of it is in the form of neutron-rich calcium-48. Producing this particular isotope is therefore time-consuming and, moreover, it costs about $200,000 per gram. As such, we have had to optimize our accelerator at JINR so that we obtain a high beam intensity for as little calcium-48 as possible.
As a target material we use neutron-enriched isotopes of plutonium (Z = 94), americium (95), curium (96) and californium (98), all of which have long lifetimes. The fusion reaction between these isotopes and calcium-48 allows us to produce elements with atomic numbers between 114 and 118, and neutron numbers between 172 and 177. This is the maximum number of neutrons possible in an element that has been produced artificially. Over a period of five years we have used a total of about 14 g of calcium-48 to create elements 112-116 and also two atoms of element 118 (see Oganessian et al. 2003 in further reading).
Experimental results
In essence, the new heavy elements with Z = 112-118 were all produced at JINR in the same way. To make element 115, for example, we used the reaction 243Am + 48Ca → 291115. The final 115 nucleus contains an odd number of protons and an odd number of neutrons, which considerably reduces the probability that it will decay via spontaneous fission. This means that there is a better chance of observing a long sequence of alpha decays that will give us information about the properties of many nuclei down to Z = 105. An even?even nucleus, on the other hand, is more likely to have short decay chains because it has a higher probability of decaying via spontaneous fission.
Before the crucial fusion reaction can take place the calcium-48 ions need to have enough energy to overcome the Coulomb barrier, which is 236 MeV for this system. However, in order to increase the probability of fusion we used a slightly higher ion energy of 248 MeV, which gave the 291115 nucleus a thermal energy of about 40 MeV.
A compound nucleus with this excitation energy de-excites by emitting three neutrons and gamma-rays to form the isotope 288115, which also has odd numbers of both protons (115) and neutrons (173). As soon as it has formed, the 288115 isotope continues to decay into lighter heavy elements. For instance, after five alpha particles have been emitted we are left with element 105, which is called dubnium.
Once it has been produced in the americium target, the heavy nucleus has a kinetic energy of about 40 MeV and flies through the gas-filled chamber of a magnetic separator. This device directs the nucleus towards the detector, while deflecting the calcium nuclei and other unwanted reaction products. After 1 μs the nucleus is stopped in the front layer of the detector, and about 80 μs later the data-acquisition system gives information on its arrival time, energy and co-ordinates.
About 20-30 s after such an event, our detector registered five further signals, the positions of which were all within 0.5 mm of the heavy nucleus. It then registered nothing until the following day, 28.7 h later, when two signals were obtained from the same position with a total energy of about 200 MeV. In other words, this was the signature of the spontaneous fission of element 105. The long lifetime of the final 105 nucleus, which has N = 163, is due to the closed neutron shell at N = 162.
Decay chains like these were registered three times in total, each of which contained six generations per family: five sequential alpha decays terminated by spontaneous fission. The energy and emission time of the alpha particles
in these three cases were strongly correlated, which means that each decay chain corresponds to the production and decay of the same element. Similar chains were observed when we synthesized the even-Z nuclei of elements 112, 114 and 116, with the lifetimes of the final nuclei in each chain ranging from minutes to hours depending on their proton and neutron numbers. In the absence of nuclear-shell structure, none of these heavy nuclei would exist for longer than about 10-19 s.
The general pattern
We now have data on the properties of 29 new nuclei with atomic numbers between 104 and 118. The decay modes, energies and lifetimes of the heaviest nuclei all agree with the predictions of the microscopic nuclear model, which provides the first experimental evidence for an island of stability in superheavy nuclei.
But we have only reached the shores of this island. We have found a steep rise in the stability of superheavy nuclei with atomic number, but we are still far from the region in which nuclei may live for thousands, maybe even millions, of years. The problem is that we do not yet know how to make the neutron-rich nuclei that will take us towards the magic number N = 184. However, there could be a way round this. If the longest living superheavy nucleus has a half-life of tens of millions years then it should be present in very small quantities on Earth. The only difficulty then is finding it.
One potential candidate for such a long-living element is hassium (Z = 108), which contains about 180 neutrons. In 2001 a team of chemists from Switzerland, Germany and Dubna found that the chemical properties of the short-lived isotope hassium-269 are very similar to those of the dense metallic element osmium (Z = 76), which lies in the same column of the periodic table. The osmium sample might therefore contain a very small quantity of the hassium isotope, which will either undergo spontaneous fission or undergo successive alpha or beta decays until its lighter daughter nuclei undergo fission.
Later this year an experiment led by the present author, in collaboration with researchers in France, will attempt to register these rare spontaneous-fission events in an osmium sample. If our experiment – which is located deep underground in Modane, France, to shield it from cosmic rays – detects just one spontaneous-fission event over a period of one year, we will know that osmium contains an extremely low concentration of element 108. As such we will have found a superheavy element that lies almost on top of the island of stability, which would allow us to test the shell model even further.
In the mean time, we will continue to search for even heavier elements at JINR. We are currently improving the sensitivity of our experiment and increasing the intensity of the beam. Ultimately, however, we will need to use a heavier projectile than calcium-48 if we want to stretch the boundaries of the periodic table even further.