Lasers have been used for the first time to create antihydrogen, which could allow precise spectroscopic measurements of anti-atoms
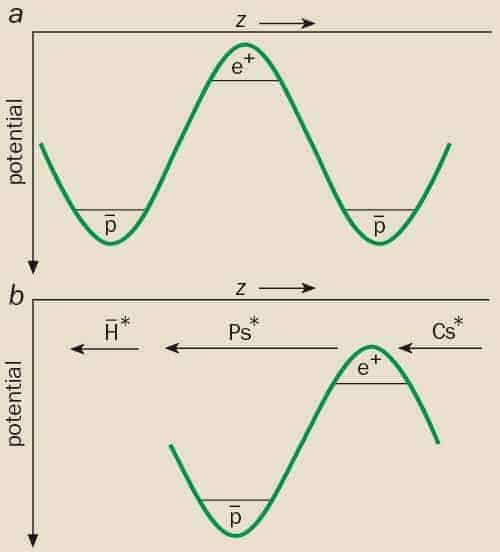
The philosopher William James once said that “if you wish to upset the law that all crows are black, you must not seek to show that none of them are – it is enough to produce a single white crow”. Likewise, if you wish to test the so-called CPT theorem, according to which a world constructed of antimatter behaves exactly the same as one constructed of matter, you do not need to create an entire “antiworld”. It would be quite sufficient to show that the frequency of just one transition in a simple anti-atom differs from the value of the same transition in the corresponding ordinary atom.
The question is, by how much? Any gross violations of the CPT theorem – which, more formally, states that a system remains unchanged under the combined operations of charge conjugation, parity reversal and time reversal – have already been ruled out experimentally. As a result, nobody expects any difference between matter and antimatter to be anything other than minute, if, indeed, there is a difference at all.
The laser-spectroscopy tools that have made it possible to measure transition frequencies in ordinary hydrogen to extraordinarily high precision should also be applicable to antihydrogen. This makes hydrogen anti-atoms excellent candidates to test the CPT theorem. Now, researchers in the ATRAP collaboration at CERN have taken an important step along the obstacle-strewn path towards this goal by using lasers to control the production of antihydrogen atoms (A H Storry et al. 2005 Phys. Rev. Lett. 93 263410).
Orbital obstacles
An antihydrogen atom consists of a positron (anti-electron) that is bound to an antiproton by the Coulomb force. Antihydrogen does not exist naturally, since it would immediately annihilate with ordinary matter. It was first produced at the PS210 experiment at CERN in 1995, but the anti-atoms formed were far too energetic to allow precise tests of the CPT theorem. This all changed in 2002, when the first “cold” antihydrogen atoms were produced by the ATHENA and ATRAP experiments, also at CERN (see Physics World October 2002 p3).
The idea behind these experiments can be likened to a space probe trying to enter into orbit around a distant planet: just as the probe has to perform some kind of manoeuvre to stop it just going around the planet and sailing out on the other side, a positron will not go into orbit around an antiproton unless it can jettison some energy and momentum as it passes. The space probe can do this by braking against the planet’s atmosphere or by burning fuel in reverse thrust. However, the positron can only do it if some third body – such as another positron or an electron – arrives just when the positron is near the antiproton to carry off the necessary energy and momentum.
The problem is that antiprotons and positrons are typically produced at speeds close to the speed of light. This means that even if they were to approach one another, they would not stay together for long enough to form a bound state, and during this short time the third body also has to come close. To produce a reasonable amount of antihydrogen the ATRAP team used a variety of decelerating and cooling techniques to reduce the relative velocity of the antiprotons and positrons from some 105 km s-1 to a fraction of 1 km s-1, corresponding to a temperature of about 4 K. The researchers then gave the positrons many chances to combine with the antiprotons by trapping both particles in an electrostatic potential well.
Since the positron has a positive electric charge, while the antiproton is negatively charged, a potential well for a positron is a potential “hill” for an antiproton. The ATRAP team therefore originally adopted a nested-trap configuration, consisting of a hill located in the middle of a well. This caused the antiprotons and positrons to settle into separate regions of the trap, so the particles then had to be coaxed into mixing with one another. It turned out, however, that this mixing procedure produced extremely hot anti-atoms, with velocities equivalent to a gas temperature of 2400 K (a kinetic energy of 0.2 eV). Moreover, being neutral these antihydrogen atoms quickly escaped from the electromagnetic trap.
This is precisely what has to be avoided for spectroscopic experiments, in which the atoms need to be held relatively still within the volume coverable by a laser beam. Magnetic “multipole fields” can do this job by producing central restoring forces on the magnetic dipole moment of antihydrogen atoms. But such magnetic traps cannot be made much deeper than about 0.5 K.
Positive solution
The solution to some of these difficulties was first proposed in 1988 by Mike Charlton, then at University College London, and Bernard Deutch of Aarhus University in Denmark. They pointed out that the positronium, which consists of an electron and a positron in orbit around one another, already carries with it the extra third body (the electron in this case) required to carry off the unwanted energy and momentum. However, since positronium is a matter-antimatter system, it rapidly self-annihilates. Worse still, it is electrically neutral, which means that it cannot be confined in a potential well. Each positronium atom will therefore only get a single shot at getting into orbit with an antiproton and making an antihydrogen atom.
By somehow increasing the radius of a positronium atom, however, these problems can be compensated for to some extent. A larger positronium atom would present a bigger “target” or cross-section to the antiproton, and the electron and positron – being further apart – would not annihilate so quickly.
The ATRAP team has now reported the first results using this new method of synthesizing antihydrogen. The researchers first accumulated some 2.5 x 104 antiprotons from the CERN Antiproton Decelerator and 1.4 x 106 positrons from a radioactive source. Then they placed the different clouds of antiparticles in adjacent potential wells that had opposite signs, rather than the nested structure of previous approaches. Both clouds were then cooled to 4.2 K using liquid helium.
The team then excited a beam of caesium atoms, the outer electron of which is bound by just 3.9 eV, with lasers at visible wavelengths. This produced caesium atoms with an outer electron bound by just 0.01 eV. When the atoms drifted through the potential well confining the positrons, these “loosened” electrons were able to jump into orbit around the positrons.
A well-known feature of such a charge-exchange reaction is a resonance effect that occurs when the electron binding energy in the final state is the same as that in the initial state. Consequently, about one in four of the positrons in the trap produced an excited positronium atom. It would require a photon with an energy of 6.79 eV to directly excite a positronium atom to such an excited state, but sufficiently powerful photon sources at this wavelength are impractical. Being neutral, the positronium atoms then drifted out of the positron well and through the adjacent well containing the antiprotons. Here, a second charge-exchange reaction produced antihydrogen atoms with the same binding energy as both the caesium and the positronium atoms (see figure).
A step closer
What are the advantages of these electromagnetic gymnastics over the original nested-trap method of producing antihydrogen? First, the equality of the binding energies in the two charge-exchange reactions ensures that the anti-atoms have little surplus kinetic energy and should emerge at a temperature of 4.2 K rather than 2500 K. Advanced cryogenic techniques could reduce this to perhaps a few hundred millikelvin in the future, enabling the anti-atoms to be enclosed and studied in a magnetic multipole well.
Second, it may also be possible to tune the principal quantum number, n, of the anti-atoms by adjusting the excitation of the caesium atoms. On the other hand, the very effect that at large values of n increases the positronium lifetime and radius, means that it would take a long time for these high-n antihydrogen atoms to decay to the ground state, which is crucial for high-precision spectroscopy. Such measurements have certainly moved a few steps closer, but it will be several more years before we see the published results in a journal.