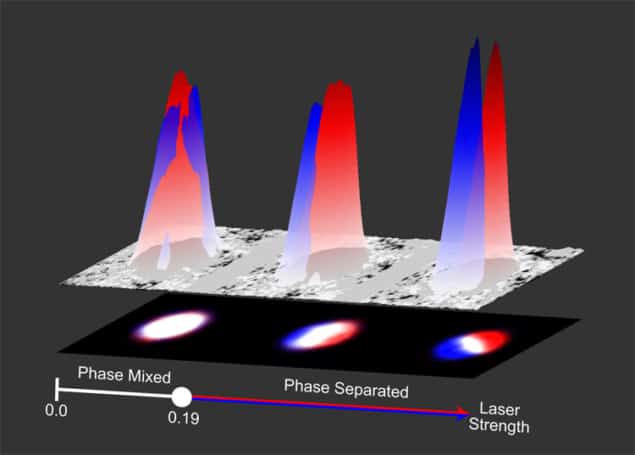
Spin-orbit coupling – a ubiquitous interaction in condensed-matter physics – has been simulated in ultracold neutral atoms for the first time by physicists in the US. Their experiment involves firing laser beams at rubidium atoms and allows the relative strength of the interaction to be adjusted by simply tweaking the intensity of the lasers. The discovery could allow ultracold atoms to be used as “quantum simulators” to investigate the role of spin-orbit coupling in a wide range of phenomena in solid semiconductors, superconductors and magnets.
For the past decade or so, physicists have been using lasers and magnetic fields to trap collections of atoms and cool them to near absolute zero. By carefully manipulating the laser light and magnetic fields, researchers can control the interactions between atoms. This allows scientists to simulate interactions that occur between electrons in solid materials in a cleaner, well controlled environment. But unlike electrons in solids, the strength of these interactions can be easily adjusted – allowing physicists to test theories of condensed-matter physics in these “quantum simulators”.
Spin-orbit coupling describes the interaction between the intrinsic spin of an electron in a solid and the magnetic field induced by the motion of electron relative to the surrounding ions. As it links the spin of the electron to its motion, spin-orbit coupling is a key parameter in the design of “spintronic” devices, which use the electron’s spin instead of its charge in circuits that could someday lead to faster and more energy efficient computers. What Ian Spielman and colleagues at the National Institute of Standards and Technology (NIST) in Maryland and the National Polytechnic Institute in Mexico City have now done is to simulate spin-orbit coupling using neutral rubidium atoms.
Rubidium clouds
The team began with a cloud of about 180,000 rubidium atoms chilled to below 100 nK to create a Bose–Einstein condensate (BEC). A BEC forms when identical atoms with integer spin are cooled until all the atoms are in the same quantum state. The team then fired two nearly identical lasers, X and Y, that intersect the BEC at a right-angles to one another. The laser light is set to resonate with a transition between two different spin states of the rubidium atom that are used to simulate the “spin-up” and “spin-down” states of the electron.
The resonance involves the atoms continuously absorbing and emitting photons. As these photons carry momentum, it means that if an atom absorbs a photon moving in the X-direction and then re-emits it in the same direction there is no change in the atom’s momentum. However, an atom absorbing a photon from the X beam can be stimulated by the Y beam to emit a photon in the Y-direction, thus changing the momentum of the atom. The result, according to Spielman, is a coupling of the spin and momentum of the atom: a spin-orbit interaction.
Tracking shadows
The team began its measurements with the lasers off and all the atoms in a spatial mixture of both spin states. The intensity of the lasers was then slowly increased, which causes transitions between the spin states and puts the BEC into a superposition of spin-up and spin-down states called a “dressed state”. Finally, the lasers and magnetic trap are switched off and the cloud of atoms is allowed to expand, with the momentum measured by shining light on the gas and tracking the resulting shadow.
The researchers then worked out the spin of the atoms by switching on a magnetic field gradient, which deflects spin-up and spin-down electrons in different directions – as in the famous Stern–Gerlach experiment. Although the atoms in the dressed state have zero net momentum, the measurements reveal that the component spin-up and spin-down states themselves have non-zero momenta. According to Spielman, this is in agreement with calculations that include spin-orbit coupling.
Surprise, surprise
The measurements also revealed two surprises. The first is that the dressed spin states are separated in space, with one spin moving to one side of the BEC and the other moving to the opposite side. Normally, it is energetically favourable for the two spins to mix in the BEC, but Spielman and colleagues believe that the lasers introduce an “exchange energy” that causes opposite spins to repel each other.
The other surprise for the team is that the spin-orbit interaction had important effects even when the laser intensity was relatively low. This means that it should be possible to simulate the interaction in an ultracold gas of fermionic atoms, which are more similar to electrons than the bosonic atoms used in this experiment. The reason, according to Spielman, is that the fermionic atoms that physicists have managed to cool (such as potassium-40) heat more rapidly when put into dressed states.
Indeed, Spielman says that his team is currently building a new experiment that uses fermions. If successful, the team should be able to induce “p-wave” coupling between fermions – an interaction that leads to superconductivity in superfluid helium-3 and some other materials. Spin-orbit coupling in fermions could also give rise to “Majorana fermions”. These are exotic particles that are identical to their antiparticles and that could be used in quantum computers.
The research is published in Nature 471 83.