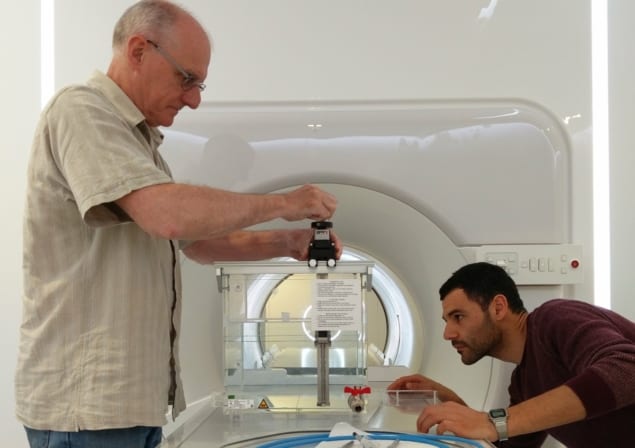
MR-guided radiotherapy enables real-time imaging during radiation delivery with high soft-tissue contrast, and could ultimately enable real-time adaptive treatments. Reference dosimetry in the presence of a strong magnetic field, however, is challenging.
“A reference dosimeter is a detector that is calibrated directly by comparison to a national dosimetry standard, or indirectly through intermediate calibrations,” explains Ilias Billas from the UK’s National Physical Laboratory (NPL). “Its main task is to enable hospital physicists to measure radiotherapy doses accurately and consistent with international dosimetry standards.”
The ionization chambers used for reference dosimetry in conventional radiotherapy systems are strongly affected by magnetic fields – making it challenging to perform beam output measurements. As such, there’s a real need for a robust and stable reference dosimeter for use in MRI-guided radiotherapy. A team headed up at NPL is investigating the suitability of an alanine detector for this task (Phys. Med. Biol. 10.1088/1361-6560/ab8148).
Dosimeter characterization
Alanine is an α-amino acid that produces a stable free radical when irradiated. The concentration of these free radicals is proportional to the absorbed dose, and is measured using electron paramagnetic resonance (EPR) spectroscopy. As alanine is a solid-state detector, it should experience a lower electron return effect (ERE) than an air-filled ionization chamber.
To quantify the performance of the alanine dosimeter in the presence of a magnetic field, Billas and co-workers performed measurements and Monte Carlo (MC) simulations of alanine pellets irradiated at three photon beam energies and various magnetic flux densities. They placed pellets (roughly 2.3 mm high and 5 mm in diameter) in a waterproof polyether ether ketone (PEEK) holder shaped like a Farmer-type ionization chamber. They then placed these alanine dosimeters in an electromagnet and irradiated them with either a 60Co source, or 6 or 8 MV linac beams, over a range of magnetic flux densities
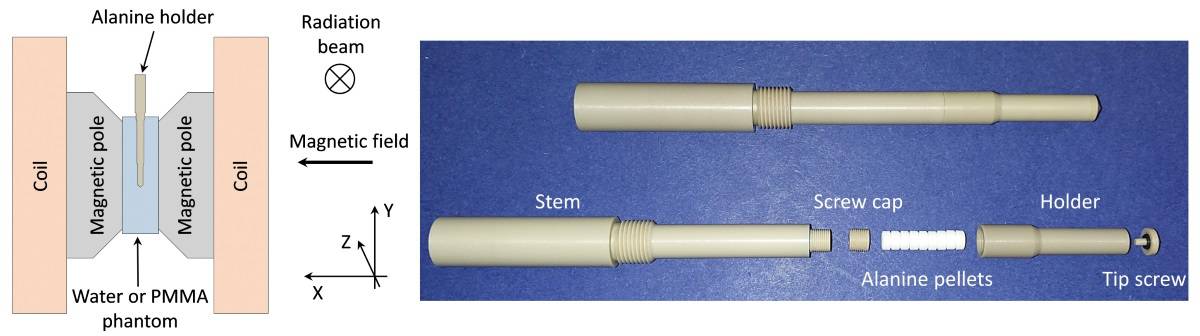
For 60Co irradiation, the researchers irradiated the alanine dosimeters inside a PMMA phantom, with any air gaps between the phantom and holder filled with water to avoid the ERE. They irradiated the phantom within magnetic flux densities of 0, 0.5, 1, 1.5 and 2 T. In the linac setup, they placed the alanine holder in a water phantom and irradiated the dosimeter at 0, 0.35, 0.5, 1 and 1.5 T.
The researchers validated MC models of the 6 and 8 MV linac beams by comparing simulated with experimental beam profiles and depth doses. To validate the model of the experimental set-up, they performed MC simulations and measurements, at 1.5 T, with the holder partially loaded with alanine pellets. In both cases, the MC models were successfully validated and used to support their research.
One potential issue with placing alanine pellets inside the holder is the impact on measured dose of air gaps within the holder – due to the bevelled edge of the pellets and the space between the pellets and the holder’s inner wall. Simulations performed at 1.5 T, with and without air gaps, revealed that such gaps did affect the alanine response, due to the ERE caused by the magnetic field. The maximum deviations between models with and without air gaps were 0.45% and 0.55%, for 6 and 8 MV beams, respectively. For the 60Co beam, all data deviated by less than 0.4%.
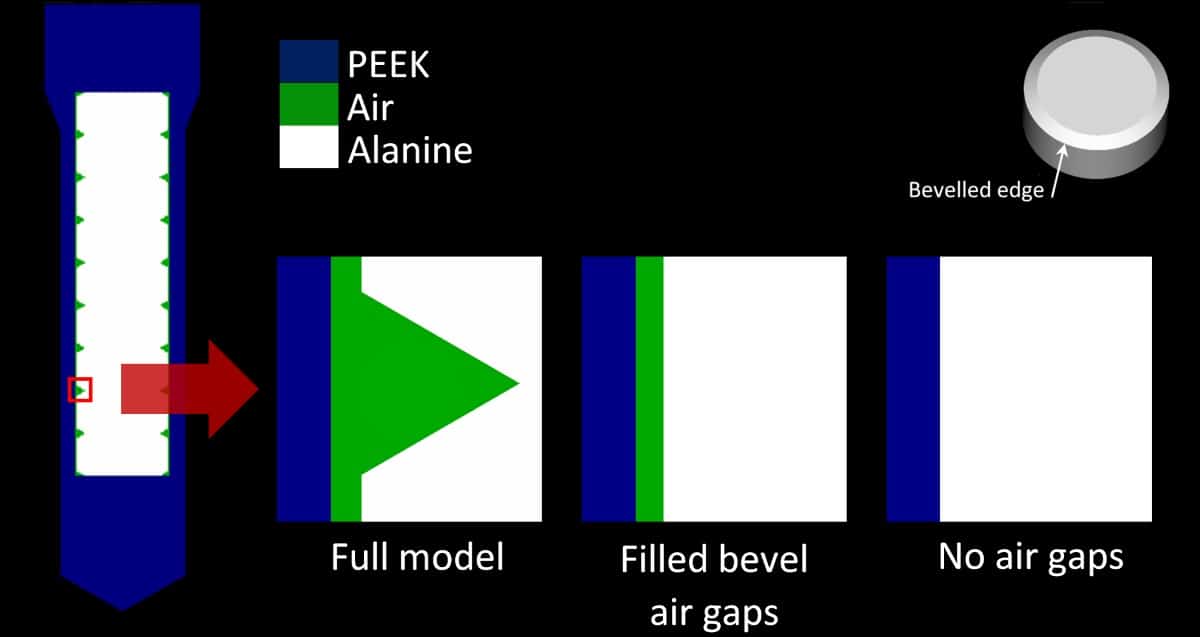
The team also investigated uncertainties due to the random positions of pellets inside the holder. MC simulations of the pellets in four different positions inside the holder showed that uncertainties increased with magnetic flux density, up to 0.52% and 0.47% for 6 and 8 MV at 1.5 T, respectively. For 60Co, the highest uncertainty was 0.52% at 2 T. For other magnetic flux densities, uncertainties were all below 0.30%. Billas notes that this is the dominant component in their uncertainty budget, which includes the unavoidable effect on the alanine response due to the air gaps.
Correction calculations
By combining their measurements with Monte Carlo simulations of absorbed dose in water, the researchers found that the response of alanine to ionizing radiation was modified in the presence of a magnetic field. The effect was energy independent and, if uncorrected, could increase the alanine/EPR signal by 0.2% at 0.35 T and 0.7% at 1.5 T.
To determine the true absorbed dose in the presence of a magnetic field, the team calculated a correction factor. This factor – which incorporates the effects of the magnetic field on intrinsic alanine sensitivity, dose distribution in water, dose to alanine and fluence perturbation by the holder – tended to decrease with increasing magnetic flux density. Averaged over all magnetic flux densities, the calculated correction factors were: 0.9946 ± 0.0019 for 60Co; 0.9973 ± 0.0018 for 6 MV beams; and 0.9982 ± 0.0033 for 8 MV beams.
The team concluded that, with inclusion of this small correction factor, alanine/EPR provides a suitable reference class detector for MRI-guided radiotherapy, with comparable uncertainties to a Farmer-type ionization chamber.
“The next step in this project is the development of guidelines for a new dosimetry calibration protocol and the development of methodologies for dosimetry audit,” says Billas. “This would involve the use of alanine to provide the traceability from the NPL’s primary standard of absorbed dose, a graphite calorimeter, from a conventional linac to an MRI-linac.”