Two new experiments have detected charge-symmetry breaking, the mechanism responsible for protons and neutrons having different masses
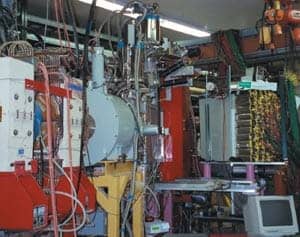
Symmetry breaking is often seen as a bad thing. The greater the asymmetry in our faces, so we are told, the less attractive we appear to others. However, if nature did not break a subtle symmetry called charge symmetry to a very small extent, the implications would be somewhat more severe. Protons and neutrons would weigh the same, and the Sun would never have shone.
Symmetry is a crucial concept in the theories that describe the subatomic world because it has an intimate connection with the laws of conservation. For example, the fact that physics is the same – or invariant – everywhere in the universe means that linear momentum is conserved. Some symmetries, such as rotational invariance, are perfect. Others, such as parity, are broken by small amounts, and the corresponding conservation law therefore only holds approximately.
The theory of the strong interaction between quarks – quantum chromodynamics – is approximately invariant under what is called charge symmetry. In other words, if we swap an up quark for a down quark, then the strong interaction will look almost the same. This symmetry is related to the concept of “isospin”, and is not the same as charge conjugation (in which a particle is replaced by its antiparticle).
Charge symmetry is broken by the competition between two different effects. The first is the small difference in mass between up and down quarks, which is about 200 times less than the mass of the proton. The second is their different electric charges. The up quark has a charge of +2/3 in units of the proton charge, while the down quark has a negative charge of -1/3.
If charge symmetry was exact, the proton and the neutron would have the same mass and they would both be electrically neutral. This is because the proton is made of two up quarks and a down quark, while the neutron comprises two downs and an up. Replacing up quarks with down quarks, and vice versa, therefore transforms a proton into a neutron. Charge-symmetry breaking causes the neutron to be about 0.1% heavier than the proton because the down quark is slightly heavier than the up quark.
The electrostatic repulsion between quarks, however, should make the proton heavier, since it contains two up quarks with charges of +2/3. This makes the electromagnetic interactions inside the proton stronger than those inside the neutron, so they should contribute to a greater mass. But the mass difference between the quarks wins over their electrostatic repulsion by a factor of about two, making it the dominant cause of charge-symmetry breaking.
Mass effect
The neutron-proton mass difference has important consequences for the structure of the universe because it means that a neutron can decay into a proton (plus an electron and an antineutrino) in radioactive beta decay. When protons and neutrons combined to form elements in the first few minutes after the Big Bang, the resulting elemental abundances depended on the neutron-proton mass difference. All the neutrons that survived were bound inside nuclei, which left many protons free. It is the interactions between these protons that are the main source of energy in stars like the Sun.
It should be possible to calculate the masses of the neutron and proton using quantum chromodynamics (QCD) and electromagnetism. But QCD is an untamed beast. The interactions between quarks are so strong that it makes calculations extremely difficult, and capturing the small effects of the up-down quark mass difference is akin to finding gold in a mountain of sand. The only way to improve the current state of our knowledge is to perform difficult experiments.
Physicists had already elucidated certain aspects of charge-symmetry breaking, but our spirits were raised greatly when we heard of the recent work of Allena Opper of Ohio University in the US and co-workers at the TRIUMF laboratory in British Columbia, Canada. Her team has been trying to observe a small charge-symmetry-breaking effect for several years, using neutron beams at the TRIUMF accelerator. The researchers studied the production of neutral π-mesons (pions) when a neutron is captured by a proton in a hydrogen target to form a deuteron. The probability, or cross-section, for this n + p → d + π0 reaction to occur depends on the angle, θ, between the momentum of the outgoing pion and that of the incident neutron beam (see figure 1).
Interchanging a neutron and a proton under charge symmetry is identical to changing the angle of the outgoing pion by 180°. The observed cross-section should therefore be symmetric about 90° if charge symmetry is an exact symmetry, which means that finding a slight asymmetry in the n + p → d + π0 cross-section would provide strong evidence for charge-symmetry breaking. But this is not easy. The TRIUMF team had to overcome the technical challenge that the pions do not have sufficient energy to escape from the hydrogen target. Instead the researchers relied on detecting the deuterons, which have more energy and do escape.
But how does the mass difference between the up and down quarks in protons and neutrons relate to neutral-pion production in the first place? The pion consists of up and down quarks and antiquarks, and its wavefunction, uu-bar-dd-bar, becomes -(uu-bar-dd-bar) under the charge-symmetry operation. In the the n + p → d + π0 reaction, charge symmetry allows the production of a similar particle to the pion called an eta-meson, η, which has a wavefunction uu-bar-dd-bar. Unlike the pion, the η wavefunction is unchanged by the charge-symmetry operation, which means that charge-symmetry breaking sometimes causes the η to transform into a π0 as it flies out of the reaction. This is the quantum-mechanical analogue of transforming an apple into a pear as it falls from a tree.
Backwards and forwards
Charge-symmetry breaking also manifests itself in the interactions of pions with protons and neutrons in a very interesting way that is linked to the neutron-proton (and hence, up and down quark) mass difference. Because the masses of the up and down quarks are almost zero, another approximate symmetry of QCD called “chiral” symmetry comes into play. This symmetry relates to the spin angular momentum of fundamental particles. Quarks can either be “right-handed” or “left-handed”, depending on whether their spin is clockwise or anticlockwise with respect to the direction they are moving in. Both of these states are treated approximately the same by QCD.
Two years ago the present authors teamed up with Jouni Niskanen from the University of Helsinki in Finland to see how the different manifestations of charge symmetry are revealed in the TRIUMF experiment. Mixing between η and π mesons causes an asymmetry in the direction of the incoming (forward) neutron beam. But we predicted that chiral symmetry would cause pion interactions to overcome the η-π mixing and generate a preference for pions to emerge in the backward direction.
We anxiously waited while the experimentalists were busy at work, and they have now just announced their results – an asymmetry in pion production of about a two parts in 1000 towards the backward direction. But the game is not over yet. The effect is smaller than we expected so there is still something that we do not understand. Enter another experimental team, led by Andy Bacher and Ed Stephenson at Indiana University in the US.
Since the 1950s experimentalists have been trying to detect the formation of a neutral pion and an alpha particle in the fusion of two deuterons, d + d → α + π0. The wavefunctions of both the deuteron and the alpha particle are invariant under the interchange of up and down quarks but recall that the pion wavefunction is not. The initial state of the reaction is therefore “even” under charge symmetry, but the final state is odd. In other words, charge symmetry would prevent this reaction from ever occurring.
Bacher and Stephenson noticed that the exquisite capabilities of the Indiana University Cyclotron Facility (IUCF) could allow a measurement of this reaction for the first time, provided it had the cross-section that we had estimated. In the IUCF set-up, a deuterium beam is focused onto a target of the same material, and the high precision of the accelerator means that the beam energy can be set at the correct energy to produce neutral pions – without producing any other particles. Sensitive detectors can track the alpha particles produced and capture the two photons that are produced when the pion subsequently decays.
The experiment was approved and everything was set and ready, except for the fact that the IUCF was already scheduled to be transformed into a materials and medical research facility. Bacher and Stephenson’s team worked frantically for two months and finally produced two separate observations of a beautiful peak at exactly the right pion energy. Their experimental cross-section is almost the same as our estimate, and this measurement of such a small charge-symmetry-breaking probability is an immense technical achievement.
Up and down
Now the ball is back in the theorists’ court. A large group, including Antonio Fonseca at the University of Lisbon in Portugal, Anders Gardestig and Chuck Horowitz at Indiana University, Andreas Nogga at the University of Arizona, and the present authors, is carrying out the task of turning the initial estimate of the cross-section of the d + d → α + π0 reaction into a reliable calculation. The same charge-symmetry-breaking mechanisms contribute to both the TRIUMF and IUCF experiments, which means that together they can provide important information on the mass difference between up and down quarks.
The origin of the quark masses is not fully understood. In the Standard Model of particle physics, the Higgs mechanism allows the generation of such masses but it cannot predict the actual mass values. This is like having a recipe to make cookies that will work with either chocolate chips or nuts. Why are the masses of the up and down quarks almost the same, and why are the masses of the other four quarks so very different? No fundamental understanding of this mass hierarchy exists. But the TRIUMF and IUCF experiments mean that nature’s violation of charge symmetry can now be used to tackle at least the up-down piece of this puzzle.