Since its discovery 100 years ago, our understanding of superconductivity has developed in a far from smooth fashion. Paul Michael Grant explains why this beautiful, elegant and profound phenomenon continues to confound and baffle condensed-matter physicists today
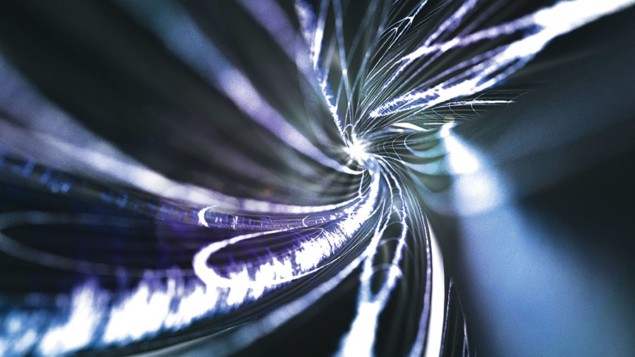
Of all the discoveries in condensed-matter physics during the 20th century, some might call superconductivity the “crown jewel”. Others might say that honour more properly belongs to semiconductors or the elucidation of the structure of DNA, given the benefits that both have brought to humanity. Yet no-one would deny that when a team led by Heike Kamerlingh Onnes stumbled across superconductivity – the absolute absence of electrical resistance – at a laboratory in Leiden, the Netherlands, 100 years ago, the scientific community was caught by complete surprise. Given that electrons usually conduct imperfectly by continually colliding with the atomic lattice through which they pass, the fact that conduction can also be perfect under the right conditions was – and is – surely no less than miraculous.
The discovery of superconductivity was the culmination of a race between Onnes and the British physicist James Dewar as they competed to reach a temperature of absolute zero using ever more complex devices to liquefy gases. Onnes won after he successfully liquefied helium by cooling it to 4.2 K, for which he was awarded the 1913 Nobel Prize for Physics. (The current low-temperature record stands at about 10–15 K, although it is of course thermodynamically impossible to ever get to absolute zero.) But researchers did not only want to reach low temperatures just for the sake of it. What also interested them was finding out how the properties of materials, particularly their electrical conductance, change under cryogenic conditions. In 1900 the German physicist Paul Drude – building on the conjectures and experiments of J J Thomson and Lord Kelvin that electricity involves the flow of tiny, discreet, charged particles – had speculated that the resistance of conductors arises from these entities bouncing inelastically off vibrating atoms.
So what would happen to the resistance of a metal immersed in the newly available liquid helium? Physicists had three main suspicions. The first was that the resistance would keep decreasing continuously towards zero. The second was that the conductivity would instead saturate at some given low value because there would always be some impurities off which electrons would scatter. Perhaps the most popular idea, however – predicted by the emerging picture of discrete, localized atomic orbitals – was that the electrons would eventually be captured, leading to an infinite resistance. But before anyone could find out for sure, researchers needed a very pure metal sample.
Gilles Holst, a research associate in Onnes’s institute at Leiden University, thought it might be possible to obtain such a sample by repeatedly distilling liquid mercury to remove the impurities that were found to dominate scattering below 10 K. The Leiden lab had lots of experience in fabricating mercury resistors for use as thermometers, and Holst suggested enclosing the mercury in a capillary tube to keep it as pure as possible before finally submersing it in a sample of liquid helium. And so it was in April 1911 (the precise date is not known for sure due to Onnes’s unclear and uncertain notebook entries) that Holst and his lab technician Gerrit Flim discovered that the resistance of liquid mercury, when cooled to 4.2 K, reached a value so small that it is impossible to measure. This phenomenon – the complete absence of electrical resistance – is the hallmark of superconductivity. Ironically, had the Leiden team simply wired up a piece of lead or solder lying around the lab – rather than using mercury – their task would have been far easier, because lead becomes superconducting at the much higher temperature of 7.2 K. In fact, three years later, acting on a suggestion by Paul Ehrenfest, researchers at the Leiden lab were able to produce and measure “persistent” currents (which would last a billion years) in a simple lead-ring sample.
History credits – erroneously in my opinion – Onnes as the sole discoverer of what he, writing in English, called “supra-conduction”. (Where the work was first published is hard to decipher, although the first report in English was in the Dutch journal Communications from the Physical Laboratory at the University of Leiden (120b 1911).) Clearly, the discovery would not have happened without Onnes, but to publish the work without his colleagues as co-authors would be unthinkable today. At the very least, the announcement should have been made under the names of Onnes and Holst. As it happens, life panned out well for Holst, who became the founding director of the Philips Research Laboratory in Eindhoven and a distinguished professor at Leiden. But that does not mean that he and others should be forgotten as we celebrate the centenary of the discovery of superconductivity.
Conforming to type
After the 1911 discovery, research into superconductivity languished for several decades, mainly because duplicating the Leiden facility was difficult and expensive. However, research also stalled because the zero-resistance state disappeared so easily when a sample was exposed to even quite modest magnetic fields. The problem was that most early superconductors were simple elemental metals – or “type I” as they are now known – in which the superconducting state exists only within a micron or so of their surface. The ease with which they became “normal” conductors dashed early dreams, voiced almost immediately by Onnes and others, that superconductivity could revolutionize the electricity grid by allowing currents to be carried without any loss of power.
However, other labs in Europe – and later in North America too – did eventually start to develop their own liquid-helium cryogenic facilities, and as the monopoly held at Leiden slowly broke, interest and progress in superconductivity resumed. In 1933 Walther Meissner and Robert Ochsenfeld observed that any magnetic field near a superconducting material was totally expelled from the sample once it had been cooled below the “transition temperature”, Tc, at which it loses all resistance. The magnetic field lines, which under normal circumstances would pass straight through the material, now have to flow around the superconductor (figure 1). This finding, which came as a total surprise, was soon followed by the observation by Willem Keesom and J Kok that the derivative of the specific heat of a superconductor jumps suddenly as the material is cooled below Tc. Nowadays observing both these bizarre effects – “flux expulsion” and the “second-order specific-heat anomaly” – is the gold standard for proving the existence of superconductivity. (Legend has it in fact that the latter measurements were actually performed by Keesom’s wife, who was also a physicist yet did not get any credit at the time.)
The mid-1930s also saw the discovery by Lev Shubnikov of superconductivity in metallic alloys – materials in which the critical magnetic field (above which superconductivity disappears) is much higher than in simple elemental metals. The experimental and theoretical study of these alloys – dubbed “type II” – quickly dominated research on superconductivity, especially in the Soviet Union under the leadership of Pyotr Kapitsa, Lev Landau and Shubnikov himself. (The latter, who was Jewish, was imprisoned in 1937 by the secret police during the Stalinist purges and later executed, in 1945.) Soviet theoretical efforts on the statistical mechanics of superconductivity – and the related phenomenon of superfluidity – continued throughout the Second World War and the Cold War, led primarily by the late Vitaly Ginzburg, Alexei Abrikosov and Lev Gor’kov. Alhough much of it was unknown to the West at the time, the Ginzburg–Landau–Abrikosov–Gor’kov, or “GLAG”, model underlies all practical applications of superconductivity. The model is so useful because it is empirical and thermodynamic in nature, and does not therefore depend on the microscopic physics underlying a particular second-order phase transition, be it magnetism, superfluidity or superconductivity.
Towards BCS theory
Progress in unravelling the fundamental theory underpinning superconductivity advanced more slowly. In 1935 Fritz and Heinz London proposed a phenomenological “adjustment” to Maxwell’s constituent equations to accommodate the notion of a “penetration depth” of an externally applied magnetic field beyond the surface of a superconductor (see “The forgotten brothers” by Stephen Blundell on page 26, print edition). However, it was not until the mid-1950s that the theoretical web surrounding superconductivity was finally unravelled, having frustrated attempts by some of the 20th century’s brightest and best physicists, including Dirac, Einstein, Feynman and Pauli. This feat was eventually accomplished by John Bardeen, Leon Cooper and Robert Schrieffer, leading to what is now called BCS theory, for which the trio shared the 1972 Nobel Prize for Physics (see “Resistance is futile” by Ted Forgan on page 33, print edition). A key development was the determination by Cooper that a gas of electrons is unstable in the presence of any infinitesimal attractive interaction, leading to pairs of electrons binding together. Bardeen and his student Schrieffer then realized that the resulting quantum state had to be macroscopic and statistical in nature.
But where did the attractive interaction come from? In 1950 Emanuel Maxwell of the US National Bureau of Standards noticed that the transition temperature of mercury shifted depending on which of its isotopes was used in the particular sample, strongly suggesting that somehow lattice vibrations, or “phonons”, are involved in superconductivity. BCS theory proved, given the right conditions, that these vibrations – which are usually the source of a metal’s intrinsic resistance – could yield the attractive interaction that allows a material to conduct without resistance.
Quite simply, BCS theory ranks among the most elegant accomplishments of condensed-matter physics. Generally stated, it describes the pairing of two fermions mediated by a boson field: any fermions, by any boson. All known superconductors follow the general recipe dictated by BCS, the basic form of which is an extraordinarily simple expression: Tc _ Θ/e1/λ, where Tc is the transition, or critical, temperature below which a material superconducts, Θ is the characteristic temperature of the boson field (the Debye temperature if it is comprised of phonons), and λ is the coupling constant of that field to fermions (electrons and/or holes in solids). A material with a large value of λ is generally a good candidate for a superconductor even if it is, counterintuitively, a “poor” metal under normal conditions with electrons continually bouncing off the vibrating crystal lattice. This explains why sodium, gold, silver and copper, despite being good metals, are not superconductors, yet lead is (figure 2).
However, BCS is descriptive and qualitative, not quantitative. Unlike Newton’s or Maxwell’s equations or the framework of semiconductor band theory, with which researchers can design bridges, circuits and chips, and be reasonably assured they will work, BCS theory is very poor at pointing out what materials to use or develop to create new superconductors. For all that its discovery was an intellectual tour de force, it is the German-born physicist Berndt Matthias who perhaps summed the theory up best when he said (in effect) that “BCS tells us everything but finds us nothing”.
Later landmarks
Following the development of BCS theory, one of the next landmarks in superconductivity was the prediction in 1962 by Brian Josephson at Cambridge University in the UK that a current could electrically tunnel across two superconductors separated by a thin insulating or normal metal barrier. This phenomenon, now known as the Josephson effect, was first observed the following year by John Rowell and Philip Anderson of Bell Laboratories, and resulted in the development of the superconducting quantum interference device, or SQUID, which can measure minute levels of magnetic field and also provide an easily replicated voltage standard for metrology labs worldwide.
For the next landmark in superconductivity, however, we had to wait more than two decades for Georg Bednorz and Alex Müller’s serendipitous observation of zero resistance at temperatures above 30 K in layered copper-oxide perovskites. Their discovery of “high-temperature superconductors” at IBM’s Zurich lab in 1986 not only led to the pair sharing the 1987 Nobel Prize for Physics but also triggered a boom in research into the field (see “Resistance is futile” by Ted Forgan on page 33, print edition). Within a year M K Wu, Paul Chu and their collaborators at the universities of Houston and Alabama had discovered that an yttrium–barium–copper-oxide compound – YBa2Cu3O6.97, also known as YBCO, although the precise stoichiometry was not known at the time – could superconduct at an astounding 93 K. As this is 16 K above the boiling point of liquid nitrogen, the discovery of these materials allowed researchers to explore for the first time applications of superconductivity using a very common and cheap cryogen. The record substantiated transition temperature rests at 138 K in fluorinated HgBa2Ca2Cu3O8+d at ambient pressure (or 166 K under a pressure of 23 GPa).
With Bednorz and Müller about to pack their bags for Stockholm as the latest researchers to win a Nobel prize for their work on superconductivity, it was a happy time for those in the field. Literally thousands of papers on superconductivity were published that year, accompanied by a now legendary, all-night celebratory session at the March 1987 meeting of the American Physical Society in New York City now dubbed “the Woodstock of physics” at which those involved, me included, had one hell of a good time.
Technology ahead of its time
Alongside these advances in the science of superconductivity have been numerous attempts to apply the phenomenon to advance old and create new technologies – ranging from the very small (for ultrafast computers) to the very large (for generating electricity). Indeed, the period from the 1970s to the mid-1980s witnessed a number of technically quite successful demonstrations of applied superconductivity in the US, Europe and Japan. In the energy sector, perhaps the most dramatic was the development between 1975 and 1985 of an AC superconducting electricity cable at the Brookhaven National Laboratory in the US, funded by the Department of Energy and the Philadelphia Electric Company. Motivated by the prospect of large-scale clusters of nuclear power plants requiring massive transmission capacity to deliver their output, the cable attracted a good deal of attention. Although the cable worked, it unfortunately turned out not to be needed as the US continued to burn coal and began to turn to natural gas. Similarly, in Japan, various firms carried out demonstrations of superconducting cables, generators and transformers, all of which proved successful from a technical point of view. These projects were generally supported by the Japanese government, which at the time was anticipating a huge surge in demand for electricity because of the country’s growing population. That demand failed to materialize, however, and I know of no major superconductivity demonstration projects in Japan today apart from the Yamanashi magnetic-levitation test track, which opened in the mid-1970s using niobium–titanium superconductors.
In 1996 I published a paper “Superconductivity and electric power: promises, promises…past, present and future” (IEEE Trans. Appl. Supercond. 7 1053), in which I foresaw a bright future for high-temperature superconductivity. A large number of successful power-equipment demonstrations once more followed, with various firms developing superconducting cables, generators, conditioners (transformers and fault-current limiters), all of which proved successful. Although few – if any – of these demonstrations have been turned into working products, there is nevertheless a lot of good, advanced superconductor technology now sitting on the shelf for the future, if needed. Unfortunately, it has so far not had much of an impact on the energy industry, which is driven as much by politics and public perception as it is by technological elegance. When it comes to the electronics industry, in contrast, price and performance – say of the latest laptop or smartphone – are everything.
A somewhat similar story accompanies the application of superconductivity to electronics, a prime example being computers based on “Josephson junctions”, which promised to bring faster CPU speeds dissipating less heat than the bipolar silicon technology that dominated from the 1960s to the early 1980s. IBM and the Japanese government bet heavily on its succeeding, as it did from a technical point of view, but were blind-sided by the emergence of metal-oxide– silicon field-effect transistors (MOSFETs), which delivered both goals without requiring cryogenic packaging. (Other applications, including my personal top five, are given in “Fantastic five” on page 23, print edition.)
Cool that sample
In January 2001, exactly a year after the dawn of the new millennium, Jun Akimitsu of Aoyama-Gakuin University in Japan announced at a conference on transition-metal oxides the discovery of superconductivity in magnesium diboride (MgB2) – a material that had first been successfully synthesized almost 50 years earlier at the California Institute of Technology. Akimitsu and colleagues had actually been looking for something else – antiferromagnetism – in this material but were surprised to find that MgB2, which has a hexagonal layered structure and can be fabricated with excellent microcrystalline detail, became superconducting at the astonishingly high temperature of 39 K. The discovery prompted many other researchers to study this simple material and, over the past decade, high-performance MgB2 wires have been fabricated. Indeed, MgB2 has the highest upper critical field (above which type II superconductivity disappears) of any material apart from YBCO, with calculations suggesting that it remains a superconductor at 4.2 K even when subjected to massive fields of 200 T.
However, there is an interesting twist to the story. In 1957 the chemists Robinson Swift and David White at Syracuse University in New York measured the lattice specific heat of MgB2 between 18 K and 305 K to see if it depended on the square of temperature, just as other layered structures do. Their results, which showed no T2 dependence, were published in the Journal of the American Chemical Society not as a graph but as a table. When their data were re-analysed after Akimitsu’s 2001 announcement and plotted in graphical form, Paul Canfield and Sergei Bud’ko at Iowa State University (as well as the present author, working independently), were surprised to find a small specific-heat anomaly near 38–39 K, indicating the onset of superconductivity.
The question is this: if the Syracuse chemists had plotted their data and shown it to their physicist colleagues, would the history of superconductivity from the mid-20th century have taken a different course? To me it is likely that all the niobium intermetallics, such as the niobium–titanium alloys used in the superconducting magnets in CERN’s Large Hadron Collider, would never have been needed, or even fully developed (figure 3). High-field magnets would have been fabricated from MgB2 and perhaps even superconducting power cables and rotating machinery made from this ordinary material would be in use today.
The lesson is clear: if you think you have a new (or old) metal with unusual structural or chemical properties, do what Holst, Bednorz and Akimitsu did – cool it down. Indeed, Claude Michel and Bernard Raveau at the University of Caen in France had made 123 stoichiometric copper-oxide perovskites four years before Chu, but having no cryogenic facilities at their lab – and, finding it awkward to obtain access to others elsewhere in the French national research council system – missed making the discovery themselves.
Superconductivity arguably ranks among the ultimate in beauty, elegance and profundity, both experimentally and theoretically, of all the advances in condensed-matter physics during the 20th century, even if it has to date yielded only a few applications that have permeated society. Nonetheless, the BCS framework that underlies superconductivity appears to reach deep into the interior of neutron stars as well, with the pairing of fermionic quarks in a gluon bosonic field experiencing a transition temperature in the range 109 K. A century after Leiden, in the words of Ella Fitzgerald, “Could you ask for anything more?”