Due to be launched by NASA this month, the GLAST satellite will study the gamma-ray sky in unprecedented detail and shed light on some of the most extreme astrophysical processes in nature, explain Julie McEnery, Steve Ritz and Neil Gehrels
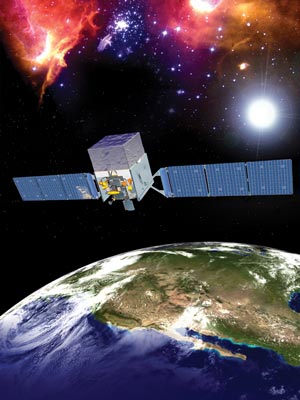
The universe appears remarkably varied when viewed at different wavelengths. The eye’s sensitivity to the visible region of the electromagnetic spectrum allows us to resolve thousands of stars, individual planets moving slowly across the sky, and even the faint diffuse glow from our own galaxy. Almost all of these photons are produced by hot, glowing objects, and by tuning instruments to higher-frequency electromagnetic radiation such as ultraviolet light and X-rays, we can explore hotter regions of the universe.
But at the highest electromagnetic frequencies — corresponding to gamma rays — the universe starts to looks more bizarre. This is because gamma rays are produced not by thermal processes but by collisions between relativistic charged particles and matter or light. Such collisions accelerate or disrupt the particles, thereby causing them to emit high-frequency photons that provide a glimpse of the most extreme astrophysical processes known.
Our understanding of these extreme environments is about to take a giant step forward. This month, NASA is set to launch its Gamma-ray Large Area Space Telescope (GLAST), a four-tonne observatory packed with state-of-the-art particle detectors that will reveal the gamma-ray universe in much richer detail. There are good reasons to expect great things.
The gamma-ray sky
Gamma-ray astronomy can be traced back 50 years to a paper by the late US theoretical astrophysicist Philip Morrison, who argued that most of the visible light upon which traditional astronomy had been based is actually “secondary” emission. For example, the Sun is powered by fusion reactions deep in its core, but the optical radiation that we see is produced not by the primary nuclear reactions themselves but by material that has been heated by them. Because these primary processes tend to occur at energies above a few mega-electron-volts, gamma-ray emission can provide a more direct indication of the underlying astrophysical processes.
It proved harder than anticipated to observe gamma rays; but in the past two decades, gamma-ray astrophysics has produced some amazing results — including the discovery of distant quasars that radiate most of their energy in the gamma-ray range. Gamma rays have a high probability of interacting with matter, which allows us to build instruments that can detect them, but these same instruments are also sensitive to large numbers of charged particles, which adds substantial background noise. Making the observational task even more difficult, gamma rays have such high energies and therefore short wavelengths that we cannot collect and focus them in the way a conventional telescope does with optical radiation. Instead, we require large and heavy instruments in order to contain the energy of the gamma rays.
The first astrophysical gamma rays were detected in the late 1960s by the Orbiting Solar Observatory (OSO-3), which observed a strong emission from the galactic plane in addition to a diffuse signal that filled the sky. In the 1970s the SAS-2 and COS-B satellite instruments, which exploited the same detection technique used in spark chambers, detected dozens of point sources of high-energy gamma rays. Then, in the 1990s, NASA’s EGRET experiment, which was operational from 1991 to 2000 on board the Compton Gamma-Ray Observatory (CGRO), revolutionized “GeV astrophysics” with the detection of several hundred gamma-ray sources.
We now know that the universe contains a rich variety of gamma-ray emitters, including pulsars, supernova remnants, gamma-ray bursts and supermassive black holes that are 106–1010 times more massive than the Sun. Even the Sun produces gamma rays by accelerating charged particles in solar flares and coronal mass ejections, while our galaxy glows brilliantly with gamma rays due to interactions of high-energy cosmic rays with interstellar gas. One of the key reasons to extend our observations of celestial gamma rays is to look for signatures of as-yet-unknown fundamental physical processes.
As gamma rays cannot penetrate even a few kilometres through the upper atmosphere, GLAST will be ideally placed to reveal the information carried by cosmic gamma rays. However, it is possible to do gamma-ray astronomy from the ground, too. When high-energy gamma rays strike matter in the atmosphere, they turn into electron–positron pairs that lose energy by emitting secondary gamma rays. This process quickly produces a “shower” of electromagnetic particles, and, provided that the energy of the initial cosmic gamma ray is greater than about 100 GeV, generates so much light that ground-based detectors — such as MAGIC in the Canaries, HESS in Namibia, VERITAS in Arizona, CANGRAOO in Australia and Milagro in New Mexico — can record them as well as the remnants of the showers.
Below energies of about 100 GeV, however, detectors must be placed above the atmosphere — and it is these energies that are of particular interest to GLAST scientists. Since the universe is transparent to gamma rays with energies below about 10 GeV, these photons allow us to study the universe at enormous distances.
In 1992 researchers at the Stanford Linear Accelerator Center and Stanford University in the US realized that advances in solid-state technology, coupled with the industry capability that had developed in anticipation of the Superconducting Super Collider, meant that they could produce an outstanding high-energy gamma-ray telescope. Following a successful NASA study showing that a large silicon-strip detector could be built for spaceflight, the mission — backed by strong support from the astrophysics community — soon became a high priority. Then, in 2000, GLAST was ranked as the highest priority mid-sized mission in a 10-year review carried out by the US National Academy of Sciences — a recommendation that boosted the telescope to the front of NASA’s queue (see Physics World April 2000 p7 print version only).
GLAST’s primary instrument, the Large Area Telescope (LAT), will cover the energy band between 20 MeV and at least 300 GeV. A second instrument, the GLAST Burst Monitor (GBM), will detect transient sources such gamma-ray bursts and solar flares down to energies of just 8 keV. In particular, GLAST will probe gamma rays in the 10–100 GeV range — a region of the electromagnetic spectrum that is largely unexplored due to limitations in the sensitivity of previous space-based gamma-ray observatories. Furthermore, the energy coverage of ground-based and space-based telescopes will overlap, thus allowing these two types of observatories to work directly together for the first time in order to cover the entire high-energy gamma-ray spectrum.
A laboratory for cosmic science
GLAST should help us to determine how much energy extreme astrophysical sources produce, and therefore tell us about the acceleration mechanisms that generate such high-energy particles. For example, pulsars, which are fast-rotating magnetic neutron stars that emit beams of radio waves from their poles, also emit lots of gamma rays. GLAST will reveal the distribution of gamma-ray energies from these ultra-dense objects, which will tell us about the geometry of the magnetic fields present and about the location of the acceleration sites. Since the large magnetic field near the surface of a pulsar can cause gamma-ray photons to be converted into electron–positron pairs, the pulsar spectra from GLAST will tell us about what the magnetic fields are like around the regions where gamma rays are emitted.
Gamma rays will also tell us about novel particle-acceleration mechanisms that are far more powerful than anything seen on Earth. Observations of supernova remnants suggest that particles can be accelerated to enormous energies by shocks produced as the blast from an exploding star ploughs into the interstellar medium. While the existence of such shocks is well established, the way in which the particles are accelerated to extreme relativistic energies is much less well understood.
GLAST’s two instruments will also provide us with the complete high-energy spectra of gamma-ray bursts, from a few kilo-electron-volts to hundreds of giga-electron-volts. These bright but distant flashes of gamma rays, which occur at a rate of about one per day, briefly shine as the brightest objects in the universe — yet the total energy released and the nature of the high-energy spectrum have never before been measured. Similarly extreme energies are produced in active galaxies when matter is accelerated to relativistic energies in a jet powered by a supermassive black hole, emitting gamma rays with a power equivalent to that of all the stars in an entire galaxy over all wavelengths. Until now, gamma-ray detectors have not been able to measure these highly variable emissions in any detail over long timescales, but GLAST will allow us to see into these jets, thus revealing their contents and dynamics.
In addition to studying these astrophysical processes, the extreme distances and energies probed by GLAST will allow us to investigate several areas in fundamental physics. One such opportunity is provided by the diffuse gamma-ray cosmic background — a haze of giga-electron-volt gamma rays that theorists currently attribute to cascades from distant tera-electron-volt gamma-ray sources, ultrahigh-energy cosmic rays, and even Hawking radiation emitted by primordial black holes, among other speculative ideas (in addition to the more prosaic theory that they originate from unresolved populations of giga-electron-volt gamma-ray sources). Thanks to its sensitivity to gamma rays with energies less than about 10 GeV, which travel across the universe unhindered because they do not lose energy by interacting with the infrared, optical and ultraviolet photons emitted by stars, GLAST will allow theorists to constrain explanations of the gamma-ray background.
The second area of basic physics that GLAST will let us study concerns one of the most fundamental questions in cosmology: the origin and distribution of dark matter. An important class of theories predicts the existence of weakly interacting massive particles, or WIMPs. In most models, WIMPs may annihilate in pairs, thus producing high-energy particles, including gamma rays. GLAST could be capable of detecting this radiation from annihilation events in the galactic halo, providing unique information about dark matter.
High expectations
Our expectations for GLAST are extremely high, based on what we have learned from previous space missions in gamma-ray astronomy. The predecessor to GLAST — NASA’s EGRET experiment — detected 271 gamma-ray sources, including over 70 active galaxies and six pulsars, thereby greatly advancing our understanding of the high-energy gamma-ray sky. But fewer than half of the EGRET sources have been definitively associated with known objects, which leaves many intriguing puzzles for GLAST to try to solve.
GLAST is designed to operate for five years and may last at least 10, complementing a new generation of ground-based detectors that have come online in the past few years. With so much new information about the universe to be revealed, and in keeping with NASA policy, all GLAST data will be publicly available. The analysis tools will also be made public, thus helping to maximize the scientific return of the mission.
We expect GLAST to have a large impact on many areas of astrophysics. Some of these have been outlined here, but what is most exciting are the surprises: with any luck, the greatest GLAST science that has not even been thought of yet.
A detector in space
GLAST, which was designed and built by an international collaboration of high-energy physicists and astrophysicists, comprises two detectors: the Large Area Telescope (LAT) and the GLAST Burst Monitor (GBM). In the former, which is a solid-state improvement on the spark-chamber technology employed by the previous EGRET detector on board NASA’s Compton Gamma-ray Observatory, gamma rays will slam into a layer of high-density material and produce an electron–positron pair. The direction of the ray can be determined by tracking the trajectories of the electron and positron using high-precision silicon-strip detectors. A separate detector, the calorimeter, counts the particles produced in the subsequent electromagnetic shower, thereby providing a measure of the initial gamma-ray energy. Surrounding the tracking detector is a layer of charged-particle sensors, the signals from which will enable gamma rays to be distinguished from the much larger “rain” of charged particles encountered in orbit. In addition to being able to detect gamma rays in the range 20 MeV–300 GeV, the LAT also has a very wide field of view: it captures about 20% of the gamma-ray sky at any time, and can survey the whole sky every three hours (two orbits). This is particularly important for observing the highly variable gamma-ray universe.
GLAST’s other main instrument, the GBM, has such a large field of view that it will be able to detect gamma-ray bursts from over two-thirds of the sky at any one time, so identifying locations for follow-up observations by the LAT. The GBM is composed of two sets of detectors: a dozen sodium-iodide sensors and two cylindrical bismuth-germanate sensors. When gamma rays interact with these crystalline detectors, they produce flashes of visible light, and the relative counting rates in the separate detectors can be used to infer the location of gamma-ray bursts across the sky. The GBM works at a lower energy range than the LAT — it can detect gamma rays down to about 8 keV — which means that together the two instruments provide one of the widest energy-detection ranges of any satellite ever built.