Without mechanical vibrations, a modern car wouldn’t get far down the road – in fact it wouldn’t start. David R Andrews examines the role of sound and ultrasound in fuel injection and parking sensors
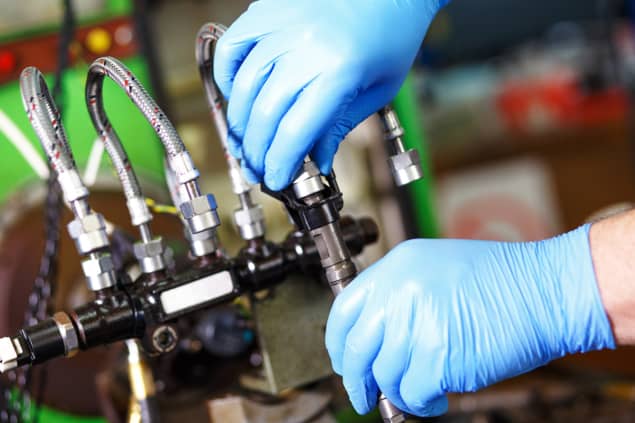
We can’t see them. In fact, we often can’t hear them either. But mechanical vibrations, whether sound or ultrasound, play a key role in modern cars. They’re perhaps most familiar in the form of those ultrasonic sensors that safely guide us into and out of tight spaces when we park or drive off. But more importantly, the vast majority of internal-combustion engines that power today’s vehicles use an adaptation of an ultrasonic transducer invented by the French physicist Paul Langevin in 1916.
Over the last 30 years about 1.5 billion road vehicles have been fitted with fuel-injection systems, of which roughly a third contain the Langevin-inspired devices. Relying on the tiny mechanical vibrations of piezoelectric crystals, they inject precise, computer-controlled amounts of fuel into an engine’s cylinders. Indeed, fuel-injection systems have been so successful that cars, lorries and trucks are no longer the polluting monsters they were 50 years ago. Today’s vehicles give off practically no lead, black soot or hazardous un-burnt petrol fumes, though they still emit lots of climate-warming carbon dioxide (CO2).
As a physicist, what fascinates me with fuel-injection systems is that they rely on piezoelectric vibrations, in this case at frequencies of 1–5 kHz. That’s well within the range of human hearing, which extends up to about 20 kHz. Above that limit lies “ultrasound”, though the precise upper frequency you can hear depends on your age and the quality of your ears. But in my profession, whether a vibration is audible sound or ultrasound is mostly immaterial: the physics is all the same. With that in mind, let’s begin our sonic car journey with a technology that’s vital before you’ve even started driving.
Sensing danger
Look closely at most modern cars and you’ll see a few small, round discs about 20 mm in diameter set into the front and rear bumpers. Hundreds of millions of vehicles have been fitted with these electro-mechanical transducers since they first entered the market in the mid-2000s. Emitting and receiving ultrasonic pulses, they’re echo-locaters, acting not only like the vocal cords of bats but also like their ears.
Pulses of ultrasound travel away from the parking transducer until they bounce off a nearby object. Some of the reflected energy returns to the transducer where it is converted into electrical signals and then sent for signal processing. The time, Δt, for a pulse to make this round trip is 2L/c where c is the speed of sound in air (roughly 330 m/s) and L is the distance to the object. With electronic digital timers able to make timing measurements of this kind very accurately (Δt ~ 300–30,000 μs), ultrasound sensors are perfect for measuring distances of a metre or two.
Simple and cheap to make, parking transducers are structured a bit like a sandwich (figure 1). Metal, usually aluminium, is first extruded to form a flat-bottomed cup with walls about 1 mm thick. A flat piezoelectric disc made from polarized lead zirconate titanate is then glued to the inside base of the cup. About 1 mm thick and 1 cm in diameter, the disc has two metal electrodes that have been evaporated onto its two flat surfaces.
The open end of the cup is sealed with a polymer layer that has two metal pins electrically wired to the two electrodes. Applying an electrical voltage to the transducer’s pins deforms the piezoelectric disc, which expands and contracts as it follows the electrical drive voltage (usually a short burst of sine waves). But there is also a smaller, radial deformation that depends on the material’s specific piezoelectric properties. With the disc glued to the inside of the device, the cup’s outer flat surface therefore buckles, creating ultrasonic vibrations at a frequency of about 40 kHz.

Sandwich transducers have many resonating frequencies, corresponding to different modes of vibration, just like the skin of a drum. Manufacturers, however, like to tune the frequency of the driving voltage so that the entire, flat surface of the transducer oscillates in phase, moving up and down like a piston. This vibration maximizes the electro-acoustic coupling, creating waves that are in phase over a wide area. In fact, because their wavelength (8 mm) roughly equals the diameter of the piezoelectric disc (10 mm), the sound waves propagate as a hemisphere with a radius expanding at the speed of sound.
Any piezoelectric transducer can also work in reverse, generating an electric charge when deformed by an incoming ultrasonic wave. Obviously, the transducer cannot transmit and receive ultrasound waves at the same time, so it receives waves only when the drive voltage is switched off (there is no timing problem because the echo arrives many microseconds later). In fact, the plane surface of the transducer is spatially matched to receive planar echo-waves, which the outgoing spherical waves will have become by the time they return.
Sandwich transducers are incredibly common as ultrasonic parking sensors, with 10 or so fitted to a typical car. They have a maximum range of about 5 m – perfect for parking – while their sealed construction means they can withstand rain and much of the grime that accumulates on road vehicles. However, their range is too short to detect other vehicles moving at speed, which is why microwave sensors or cameras are used instead for collision-avoidance purposes.
One unexpected benefit of ultrasonic sandwich transducers is that they can ward off rodents, which love nothing better than climbing into car engine bays and gnawing at tasty electrical cables and rubber hoses. Rats can hear ultrasound up to 60 kHz, while mice have ears that operate up to 100 kHz, making ultrasound transducers installed under your bonnet the ideal, low-cost rodent-scarer. The animals hear the sound, but we don’t. And you don’t even need to measure the return signals that well: all you need is a loud, intermittent ultrasound and the rodents will leave your cherished vehicle alone.
Injection of interest
So let’s assume those ultrasound parking sensors have helped you negotiate your way out of that tight parking space and you’re ready to hit the road. To get moving, you press your foot down on the accelerator pedal, which sends a signal to the car to start burning more fuel. But for it to combust as efficiently and as effectively as possible, the fuel has to be mixed with just the right amount of air.
In an internal combustion engine, this was originally done with a carburettor – essentially a pipe through which air passes into the engine. Fuel enters from the side, with a “float valve” controlling how much gets in. Problem is, the valve has to be correctly oriented with respect to gravity. Turn it upside-down and it won’t work at all. Corner your car too fast and the float valve can be disturbed by strong inertial forces, potentially starving the engine of fuel and preventing it from generating mechanical power.
This drawback of carburettors applies to aircraft engines too, as Britain’s Royal Air Force found to its cost during the Second World War. Its Spitfire and Hurricane planes were fitted with Merlin engines, which had carburettors that were prone to cutting out when the pilots performed steep dives. The German Messerschmitt 109, in contrast, used one of the first fuel-injection systems and could perform dives and manoeuvres under full power. It was a superior fighting aircraft, though in the end Britain’s ability to build new planes faster than the Germans gave Britain the decisive military edge.
Since they entered the car market in the 1970s and 1980s, fuel-injectors have transformed how we drive. With a traditional carburettor, a classic four-stroke internal combustion engine works by the piston first moving down as air and fuel enters the cylinder. On the second stroke, the piston moves up to squeeze the mixture, which ignites (spontaneously in a diesel engine; with spark plugs in a petrol engine). The burning fuel then pushes the piston back down again. Finally, the piston moves back up and expels the burnt gases, each stroke taking half a revolution of the engine’s main crank-shaft.
But with individual fuel-injectors for each cylinder, the four strokes are slightly different. On the first, the piston moves down as air (rather than an air/fuel mixture) enters the cylinder. On the second stroke, the air is compressed and only now – towards the end of this stroke – is the fuel injected. Third, the mixture burns, pushing the cylinder down. Finally the piston moves back up as the gases are expelled. By injecting fuel towards the end of the second stroke, the engine becomes both more efficient and able to run at a lower temperature.
Burning advantages
Driven by increasingly stringent emissions regulations over the last 50 years, fuel-injection systems have now largely replaced carburettors in most road vehicles. Key to their operation are tiny piezoelectric transducers, which open and close the valve to let precise amounts of fuel into the engine’s cylinders. Compared to old-fashioned carburettor engines, these fuel-injection systems allow modern cars to run much efficiently and with far less fuel for the same power output.
However, manufacturers can’t use the sandwich transducers found in the humble ultrasound parking sensor: they can’t generate displacements of more than 0.01 μm in air and are unable to work with liquid fuel, which is far harder to compress than air. Instead, most high-power ultrasonic transducers in fuel-injection systems are based on the Langevin transducer, which was invented during the First World War to help the French navy track down enemy submarines by detecting the echoes from the transducer itself.
Rather than having a single piezoelectric disc as with the ultrasonic parking sensors, a modern Langevin transducer uses a stack of them sandwiched between two metal rods. Roughly 5 mm thick and 30 mm in diameter, each lead-zirconate-titanate disc has a cylindrical 10 mm hole in the centre, through which a threaded metal screw passes. The screw holds the assembly into a single mechanical unit, which vibrates by expanding and contracting along its length (figure 2).

The Langevin transducer does two main things in a fuel-injection system. First, it actuates a “pintle valve” that allows fuel into a small chamber near to the nozzle at the tip of the injector. Second, it pumps the fuel out of the small chamber into the car engine’s cylinder at high pressure. In fact, the transducer is clamped at the end farthest from the injection nozzle by the outside body of the injector, meaning one end of the stack is stationary but the other end moves.
Manufacturers want is to maximize displacement at the tip so that fuel can be pumped into the engine as effectively as possible. We know that the displacement of the piezoelectric material is proportional to the strain, which depends largely on the applied electric field, E, across each disc. So to maximize the displacement we need to maximize E = V/d, where V is the voltage across each disc and d is the thickness of the disc. The best solution is to use lots of thin discs rather than one, single fat disc even if it is of the same overall thickness. The multiple thin discs will give the same total deformation but with a fraction of the voltage, which is handy as smaller voltages are easier to generate, safer to use and require thinner insulation.
In practice, most fuel-injectors have between 20 and 100 piezoelectric discs in a stack, with each disc being 10–20 mm in diameter and just 0.5 mm thick. They can achieve displacements of about 10 μm with drive voltages of about 150 V and boast a relatively low mechanical “quality factor”, which means they do not resonate and you can get a single mechanical pulse rather than a packet of pulses as you would with a conventional Langevin stack.
In simple systems, cars use just one injector to fire fuel into the air-intake manifold, ahead of the air-intake valves for each cylinder, thereby directly replacing a carburettor. But many cars now have one injector per cylinder, each delivering a precisely optimized amount of fuel to each cylinder to suit the quantity of air received. Nearly all the fuel gets burnt in each power stroke of the piston – practically eliminating the carbon deposits that would build up were combustion imperfect – and ensuring almost no micro-particles of carbon leave the exhaust.
As for how quickly a fuel injector needs to work, an engine running at top speed turns at about 6000 revolutions per minute, which is 100 revolutions per second. The injector therefore has to open and close in less than half a cycle of the piston, which would be 5 ms in this example, though typical opening and closing times are usually 1 ms or less. Conventional Langevin transducers, in contrast, operate at 25–60 kHz and so have a period of oscillation of 15–40 μs, which is much less than the 1 ms needed for fuel injection.
One final trick of a Langevin transducer is that it converts incoming low-pressure fuel into liquid at high pressure. This not only overcomes the compression pressure in the cylinder but also turns the fuel into a mist of fine droplets roughly 10 μm in diameter. Much more of the fuel surface is therefore exposed to air, allowing combustion to be more fully completed. The Langevin design does this thanks to its vibrations having a high “acoustic impedance” (density × wave speed).
Fuel for thought
When it comes to delivering fuel to your car engine, fuel injectors can adjust the precise amount in three ways. First, they control how long the injector is open. This method is more effective when an engine is running faster (at low speeds, where strong acceleration is needed, the fuel-pumping action cannot sustain a high pressure of fuel for the entire time the injector valve is open). Second, fuel injectors can control the voltage to the piezoelectric stack of discs (the peak voltage is about 150 V but lower values will narrow the nozzle’s aperture, thereby restricting the quantity of fuel entering the cylinder). Finally, fuel injectors can adjust fuel levels by repeatedly pulsing the voltage so the nozzle opens and closes repeatedly within one stroke of the piston.
As a pulsed system, fuel injectors therefore allow the modern internal combustion engine to be highly controlled. Indeed, some Formula 1 car manufacturers like to show how well they can do this by running their engines without a load on a test bed and programming them to play tunes such as “God Save the Queen”. The digital control of the engine, provided by the fuel-injectors, allows the engine speed – and hence the audible musical note heard from the engine – to be synthesized precisely. It’s a gimmick, but impressive nonetheless.
In reality, your car has its own computer system to calculate how much fuel to inject – and when. Performing simple tasks quickly, the “engine-control unit” collects data from several sensors on the engine and exhaust as well as the accelerator pedal, which it then compares with a table of values it holds to find what fuel-injector settings to use. Manufacturers can therefore precisely control their car engines to give drivers a choice of operation – such as economy, sport, race-track, engine longevity and low-pollution – or to meet specific pollution-monitoring tests.

Clearly, some of these modes conflict with one another or are even mutually exclusive. Economy, for example, does not fit well with sport or race-track modes, while the desire to maximize performance while beating pollution-monitoring tests can also conflict, as one large car manufacturer (Volkswagen) has found to its cost. But we should not lose sight of the fact that fuel-injection systems have transformed motoring.
Apart from letting internal-combustion engines run more efficiently and more reliably, they use less fuel and cut pollution compared with carburettors. They also let engines last longer and – by lowering the amount of pollution from exhaust gases – extend the lifetime of catalytic converters. Fuel-injection systems have not, however, cut CO2 emissions from road vehicles and surely the future of road transport will involve burning hydrogen in internal combustion engines (producing water instead of CO2) or switching wholesale to electric vehicles.
Both changes would eliminate CO2 generation, provided that the hydrogen and electricity in each case can be supplied without emitting its own greenhouse gases. In the meantime, however, we can thank the vibrations of tiny piezoelectric crystals for at least helping us to burn fuel better. In fact, more small-particle pollution comes from the action of car brakes and tyres than from the exhaust fumes of current internal-combustion engines – a testament to the effectiveness of catalytic converters and to modern fuel-injection systems. Those vibrations may be small, but their benefits are big.