Note added 2 December 2003. This article received a Special Commendation in the General Medical category of the Medical Journalism Awards 2003.
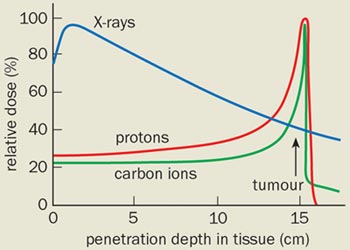
Until a cure for cancer is found, about one in three of us will probably have to undergo surgery, chemotherapy or radiotherapy at some point in our lives. Traditional radiotherapy uses X-rays to target cancerous tissue, but there is growing interest in using particles instead. Beams of hadrons, such as protons, neutrons and ions, offer important advantages over X-ray radiotherapy. Their clinical applications, however, are much less widespread.
Most of the 25 or so hadron-therapy facilities that are currently in operation around the world are sited at large particle-physics laboratories – not hospitals. A major focus of the hadron-therapy session at the World Congress in Medical Physics and Bioengineering, which is taking place in Sydney this month, will be on efforts to transform particle therapy into a practical and affordable treatment option.
Particle therapy works by damaging the DNA of cancerous cells – mostly by ionization – so that they cannot grow and multiply, while minimizing damage to surrounding healthy tissue. This is the same basic principle behind X-ray therapy (see “X-rays pinpoint tumour targets”). However, photons lose a significant amount of their energy before they reach the tumour, which can damage healthy cells and cause unpleasant side effects.
On the other hand, when a beam of charged particles enters the body, it deposits most of its energy at a depth that depends precisely on the energy of the particles. This means that tumours can be targeted more accurately, allowing a larger radiation dose to be delivered and speeding up the treatment programme.
“There is no justification for irradiating disease-free tissue if it is not absolutely necessary,” says Al Smith of the M D Anderson Cancer Center at the University of Texas, who is chairing a session devoted to proton therapy at Sydney. “There is a growing consensus that protons offer the potential for superior clinical results over photons – especially in reducing ‘late’ effects such as secondary tumours, which are caused by the treatment itself.” The trouble is that while X-rays are cheap and easy to produce, particle beams are not.
Proton therapy
The most widely used form of particle therapy is proton therapy, which was proposed by Robert Wilson – the first director of Fermilab in the US – in 1946. The first proton therapy was carried out in 1954 with the Bevatron accelerator at Berkeley, and to date about 35,000 patients around the world have undergone the treatment, mostly for cancers of the retina. Over 9000 of these were treated over the course of 30 years at the Harvard Cyclotron Laboratory in Massachusetts. The programme was transferred to the Massachusetts General Hospital in Boston last year, making it the world’s second hospital-based proton-therapy centre.
Protons, like all charged particles, slow down as they travel through a material as a result of electromagnetic interactions. The slower they move, the more efficient they are at ionizing atoms in their path and the more likely they are to interact with atomic nuclei. This means that the highest radiation dose is delivered at the point in the body at which they stop – the so-called Bragg peak – while the dose elsewhere is low (see figure 1). The ratio of radiation deposited at the tumour site to that in the path of the particle is called the relative biological effectiveness (RBE), which is basically a measure of the benefit versus risk of radiotherapy techniques.
Before treating a patient, radiation oncologists have to identify the precise location of the tumour by imaging it. This is often done using another physics-based technique – positron emission tomography (PET). The energy of the proton beam – which is generally between about 100 and 200 MeV – is then adjusted to match the tumour depth. Moreover, by combining protons with different energies in a single beam, the Bragg peak can be modulated into a plateau that dumps a high dose of radiation throughout the depth of the tumour.
There are currently six proton-therapy facilities under construction worldwide and a further 20 have been proposed, many of which will be in the US. This explosion of interest is partly due to the success of the first hospital-based proton facility at Loma Linda University in California – which has treated over 8000 patients since it opened in 1990 – and the fact that medical-insurance companies now include proton therapy as a reimbursable treatment programme.
But the technique still has some way to go before it becomes a routine service. According to Al Smith, much current research is focused on the use of lasers to accelerate protons, rather than using existing cyclotron and synchrotron sources.
“Laser-based devices can be made small enough so that each treatment room could have its own proton source,” he says. “They also offer the most substantial reduction in cost, but it is unlikely that laser proton accelerators will become a reality in the next 10 years.”
Heavy treatment
Newer than protons on the particle-therapy scene is ion therapy. Heavy ions, such as carbon, have a higher RBE than protons and are thought to provide more effective treatment for certain, deep-seated tumours that are often “radioresistant”.
This is because the rate at which a charged particle loses energy in a material – which is quantified by its linear energy transfer (LET) – increases with the mass of the particle. Helium ions were used to treat over 2000 patients at the Bevelac accelerator at Berkeley from 1957 and 1992, while neon ions have been used to treat a further 430. However, the optimal RBE using ions has since been found to lie in the range between lithium and carbon. There are currently just three heavy-ion treatment facilities in the world – two in Japan and one in Germany – all of which use carbon ions.
“It is easy to use protons, but heavy ions are superior for very resistant cancer cells,” says Kanai Tatsuaki of the Heavy Ion Medical Accelerator (HIMAC) in Japan, which has treated over 1500 patients with carbon ions since 1994. “However, many people think that heavy ions can damage healthy tissue and we are trying to demonstrate its clinical effectiveness.” Significant advances in improving the precision of carbon-ion beam delivery have been made at the GSI laboratory in Germany, where 150 patients have undergone carbon-ion therapy since 1997.
The third carbon-ion facility opened last year in Hyogo, Japan, and further facilities are planned by the TERA foundation in Italy and the University of Heidelberg in Germany. These projects have benefited from the proton ion medical machine study (PIMMS), which was based at the CERN particle-physics lab in Geneva (see Physics World June 2000 p9, print version).
Particles take charge
Charged particles such as protons and ions are not the only way to tackle tumours – neutral particles can be even more effective. Fast neutrons lose energy in the tumour via interactions with nuclei, rather than ionization, which causes cell damage that the body cannot repair. Neutrons therefore have a higher linear energy transfer than charged particles, and can produce a RBE that is up to three times that of protons or ions.
In the 1970s accelerator physicists at Fermilab realized that their linear accelerator could produce more protons than they needed, which could be used to generate a neutron beam. Funds were later secured to investigate a dedicated facility for fast-neutron therapy, which has since treated more than 3000 patients. Several other neutron facilities are currently in operation worldwide, but Arlene Lennox of the Fermilab facility thinks that neutron therapy needs more resources for outreach and marketing activities. “Fundamental science is not the issue here,” she says. “Most physicians and patients know nothing about the advantages of fast-neutron therapy; the main reason why people know about proton therapy is that Loma Linda University has been aggressively marketing it for over 10 years.”
A newer form of particle therapy that gives a high linear energy transfer is internal hadron therapy. In boron neutron-capture therapy, for example, a boron compound is first injected into the patient, which accumulates in the cancerous tissue. A beam of slow (thermal) neutrons from either a reactor or accelerator is then directed towards the tumour, where the boron atoms are split into lithium ions and alpha particles. These charged particles then destroy nearby cells but they only have enough energy to do so in a localized region, thereby sparing the surrounding healthy tissue.
“The World Congress is the first time that external-beam and internal-hadron therapies are being discussed together,” says Barry Allen of George Hospital Cancer Care Centre in Kogarah, Australia, and co-organizer of the Sydney meeting. “Internal hadron therapy offers systemic rather than local treatment, which is the key to tackling cancer.”
While still at a very early stage, a small company in the US is turning to antimatter as a potential form of particle therapy. PBar Medical is currently using antiproton beams at CERN to investigate the effects of antiprotons in biological material (see Physics World March 2003 p12, print version). Antiprotons exhibit the same Bragg peak as protons, but when they meet protons and neutrons at the treatment site, they annihilate to produce gamma rays and high energy particles that cause further damage to cancerous cells. Antiprotons might therefore have a RBE that is twice as great as protons, although this remains to be demonstrated.