The story behind a late-2016 shutdown of several reactors in France is a classic example of why materials-science research continues to be vital for the nuclear industry, as Karl Whittle, Mahmoud Mostafavi and Philip D Edmondson explain
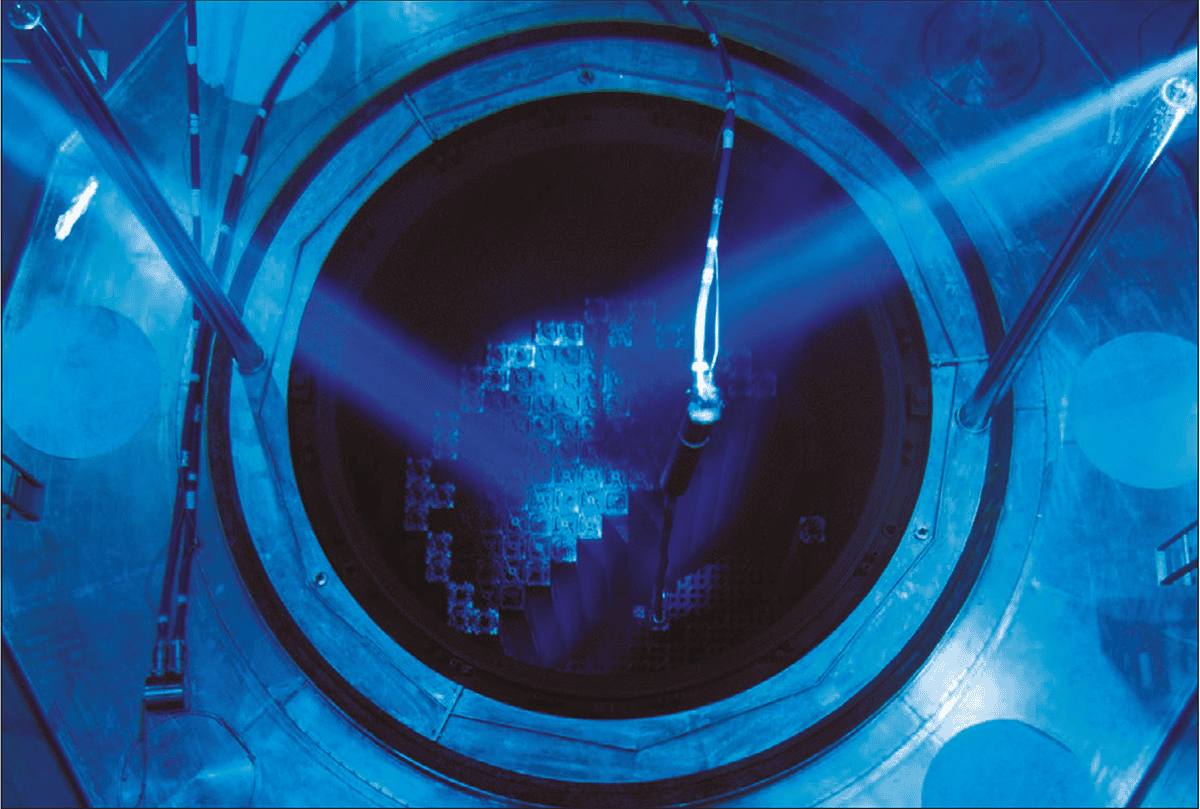
For a few weeks in the autumn of 2016, France had to do without around a third of its nuclear reactors. Several of the closures were due to routine maintenance, but the ones that hit the headlines had a rather more serious cause: quality-control problems in the manufacture of various critical reactor components.
Suspicions – and eyebrows – had been raised among industry insiders more than a year earlier when, in April 2015, the French nuclear regulator released information concerning the discovery of compositional variances in the steel used at Flamanville 3, an Areva-designed European pressurized-water reactor (EPR) on the country’s northern coast. These variances were in the head and bottom of the reactor pressure vessel (RPV), and they arose from an increase in carbon content compared to the designed standard. Following this report, further concerns were raised over other components, such as steam generators, that had been manufactured at the same facility at a similar time. These components were then examined as part of a large shutdown programme aimed at determining whether zones of high carbon concentration could have made the components less mechanically tough than they should have been – perhaps decreasing their resistance to crack propagation.
Clearly, cracks in reactor vessels are unwelcome. However, to understand fully why the French shutdown occurred, it is first necessary to consider the conditions within the core of a reactor like the EPR. Generally, within a pressurized water reactor (PWR) such as the EPRs being built at Flamanville (and, soon, at Hinkley Point C in the UK), the water temperature is about 320 °C, while pressures of around 150 bar ensure the water remains liquid and does not boil. This high-temperature water is then used to heat a secondary water circuit, creating the steam that drives the turbines. Such conditions would, by their very nature, be considered extreme, even before one includes the added complexity arising from radiation.
In such an environment, there are multiple ways for cracks to begin forming. One significant cause is a pre-existing or “locked in” point of stress or strain, which may have formed during manufacture of the component itself, or (as in this case), during the fabrication of the material that makes up the component. Cracking reduces such stress/strain, lowering the overall energy of the system and thus stabilizing the material. Another important crack-formation mechanism, stress corrosion, also reduces the stress/strain on the material, but in this case the process occurs near the surface of the material.
Both of these mechanisms constitute classical materials behaviour, and are not specific to nuclear applications. Stress corrosion can, however, be accelerated through radiolysis of the circulating water, which occurs when high-energy photons are released during fission/decay and go on to create radicals. A third important crack-formation mechanism, neutron-induced damage, is directly related to radiation. In some cases, radiation can cause alloying elements or impurities to segregate in certain areas of the material, weakening it. This type of damage is a key limiting factor in the lifetime of nuclear materials, and it affects every component used within the core – whether it be fuel, cladding or, as at Flamanville 3, the RPV.
Predicting behaviour
If materials are placed under great strain within the core, what is the best way of predicting their behaviour? In many cases, the level of predictability required depends on the material’s location. For example, to predict the behaviour of the fuel-cladding interface, one must have a very good understanding of how both fuel and cladding behave during operation. Such behaviour is directly linked to fuel changes arising from fission-induced damage and thermal-induced expansion in both the fuel and cladding, coupled with fission-induced expansion of the fuel pellets. In essence, predicting this behaviour relies on taking classical materials science and augmenting it with radiation-induced changes.
The codes take into account a wide range of material properties and use them to predict long-term behaviour, including crack propagation
To say that it is difficult to routinely monitor components during their operational use within a core is something of an understatement. The levels of radiation during operation are extreme, and in many cases gaining access to components can be a challenge. For this reason, analytical codes have been developed to model component behaviour. These codes take into account a wide range of material properties (such as hardness, toughness and ductility) and use them to predict long-term behaviour, including crack propagation.
The UK’s Office of Nuclear Regulation (ONR) requires that for every reactor it approves, there must be an accepted method for predicting its expected behaviour over time, and this method must be both reliable and valid. For example, codes known as R5 and R6 are used to predict the behaviour of the advanced gas-cooled reactor (AGR) fleet. These codes are continuously updated and tested against the behaviour of real materials, thanks to a programme of monitoring and assessment that is incorporated into the maintenance and refuelling programme for each reactor. They are thus reliable models that we can use to predict how a material will behave with confidence.
The next generation
As we move into the next generation of reactors and beyond, however, the situation changes somewhat. For traditional light-water reactor designs such as a PWR, there is a breadth of experience worldwide that can help us predict long-term material behaviour in new PWRs. However, the next generation of reactors – the so-called generation-IV family – are based on very different technologies, and feature innovations such as very high temperatures (up to 850–950 °C with helium-gas coolant) or molten salt- and liquid metal-based cores. Data on such systems is sparse. Next-generation designs also have longer planned lifetimes than the current fleet, and can thus be expected to suffer higher levels of radiation damage before they are decommissioned.
Understanding and predicting how materials will behave over extended periods of time is of paramount importance
Under these constraints, understanding and predicting how materials will behave over extended periods of time is of paramount importance. Before we discuss how to handle such challenges, we need to define a unit that is both simple and powerful in its use: displacements per atom, or dpa. This, as the name implies, is the average number of atomic displacements within a material sample; a value of 1 dpa might imply that in a system of 100 atoms, each atom has moved once, or it could mean that one atom has moved 100 times. As such, it is a useful measure of the average level of damage within a system.
For a light-water reactor, the expected level of damage within the core is about 1 dpa per year of operational life. Hence, in the expected 60–70 year lifetime of reactors currently under construction, we can anticipate reaching values of 60–70 dpa. However, generation-IV designs can have damage levels greater than 200 dpa – a significant increase that dramatically complicates the information required to develop future assessment codes. Before we can trust models predicting behaviour at such high damage levels, we need to test those models against sample materials that have been irradiated to similar levels. This type of damage can be induced using a range of methods, such as bombardment with neutrons or ions. The sample must then be analysed and any changes to its properties (such as the fracture toughness) determined. This behaviour can then be included in the relevant codes.
Quality assessment
So how does this discussion of next-generation technologies relate to the increased carbon content in the RPV steel at Flamanville? The answer is that the increased carbon content took the material into a regime that was outside the design and regulatory specifications, introducing property changes that were not included in the original analysis and predictions. As a consequence, these predictions became unreliable. Further testing, requested by the French nuclear regulator, should show whether the models’ predictions are still valid, and thus whether they can be used to make future predictions for the vessels being monitored.
This incident therefore highlights the need for materials and components to be manufactured to a high degree of reliability and accuracy – not only in their shape, but also in their composition, as even small changes can lead to components falling outside of specification. Once a component is shown to be outside of specification, the regulator is well within its rights to initiate a shutdown of the reactor, and to require further testing/data before restart. Such was the case with the French reactors in 2016, when increased carbon content in the RPV steel led to a shutdown of a large portion of the nation’s fleet.