Nanofluidics could be used to purify water, generate energy and build nanoscale machines. But when water flows through a carbon nanotube, classical fluid mechanics breaks down, leading to puzzling experimental findings that researchers have attributed to an effect called “quantum friction”, as Philip Ball explains
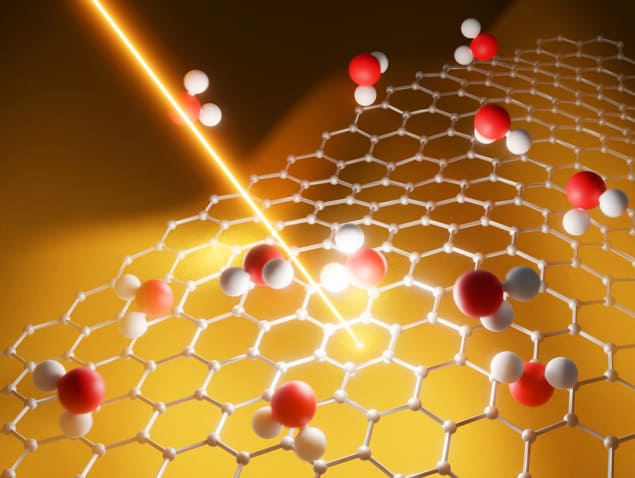
If you’re standing under a trickling shower lamenting your low water pressure, a back-of-the-envelope calculation will give you the relationship between water viscosity, pressure and the size of your water pipes. If your pipes were scaled down to a few microns wide, you’d also need to know how much friction there is between the water and the pipe itself, which becomes significant at the microscale.
But what would happen if your pipes were so narrow that only a few water molecules could fit through at once? While nanoscale plumbing might sound both impractical and impossible, it’s something we can actually build thanks to carbon nanotubes. Soon after Japanese physicist Sumio Iijima discovered multi-walled carbon nanotubes in 1991 (Nature 354 56), researchers began to wonder if these tiny structures could be used as molecular-scale tubes to suck up and transport liquids.
Carbon nanotubes have walls that repel water, leading scientists to suppose that water might zip through these structures almost friction-free. With such efficient flow, there was talk of using the nanotubes for water desalination, water purification and other “nanofluidic” technologies.
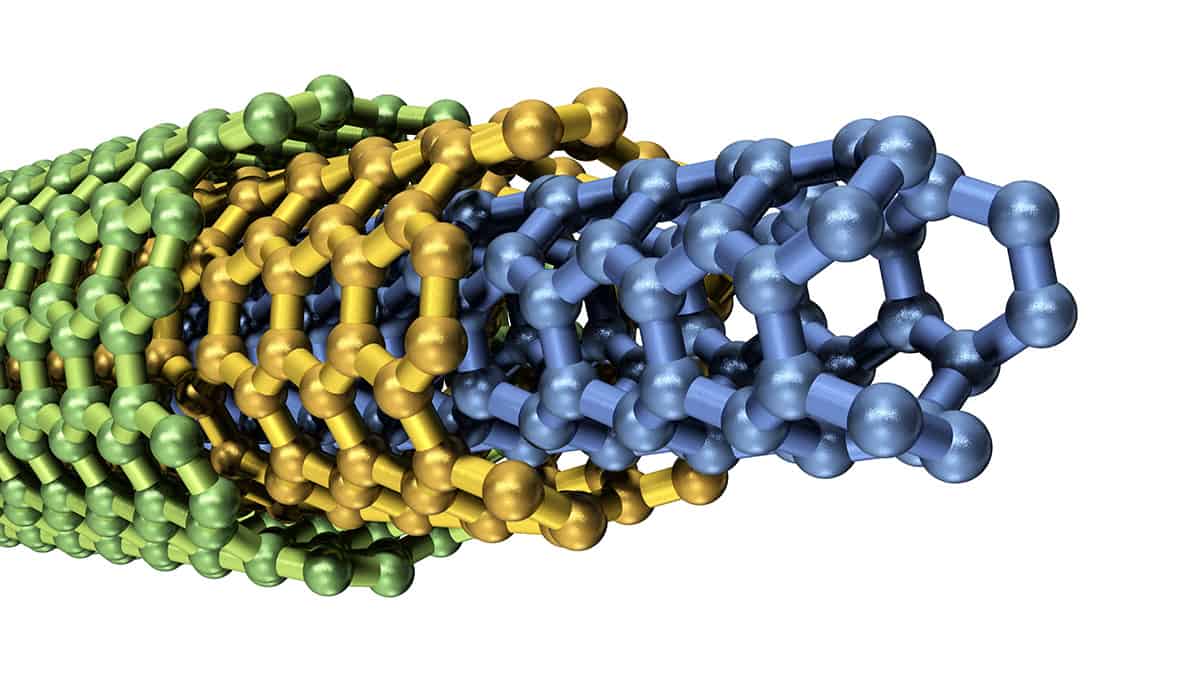
According to standard fluid dynamics, the friction between a flowing liquid and the pipe wall shouldn’t change as the pipe gets narrower. However, experiments have shown that when water flows through a carbon nanotube, the slipperiness of the tube depends on its diameter.
It turns out that at the nanoscale, the laws of fluid mechanics are governed by the quantum-mechanical aspects of the interactions between water and carbon
It turns out that at the nanoscale, the laws of fluid mechanics are governed by the quantum-mechanical aspects of the interactions between water and carbon, and can give rise to a new phenomenon dubbed “quantum friction”. Friction is often a nuisance, but whether it’s a problem or an opportunity here depends on our ingenuity.
Quantum friction might be exploited to develop nanoscale flow sensors or to make ultra-tiny valves for nanofluidics. The discovery of this surprising quantum effect – which even works at room temperature – has opened up a toybox for practical nanotechnology applications and theoretical molecular physics alike. For “quantum plumbers”, we’re only at the beginning of finding out what’s inside.
Slippery tubes
The story begins in earnest in the early 2000s, when computer simulations of water flowing through carbon nanotubes (Nature 438 44 and Nature 414 188) showed that water molecules indeed move with very low friction past the tube wall. This creates impressive flow rates, even faster than through the specialized nanoscale protein channels that regulate water levels in animal and plant cells.
Other simulations, carried out by Ben Corry at the Australian National University, suggested that if the nanotubes are only a few ångstroms across – so that just a few water molecules fit within the diameter – the structures could filter out salts (J. Phys. Chem. B 112 1427). That’s because dissolved salt ions are surrounded by a “hydration shell” of water molecules, which should be too big to pass through the tube. This finding raised the possibility of creating desalination membranes from arrays of aligned nanotubes, with the low friction ensuring high water flow rates.
Early experiments on such membranes (Science 312 1034) in the 2000s by Olgica Bakajin’s group at the Lawrence Livermore National Laboratory in California showed promise (figure 1). But the practicalities of fabricating robust, cost-effective membranes with nanotubes that are all the same size have led to rather slow progress.
1 Need for speed
The hydrophobic surface of graphene makes it an attractive material for low-friction nanoscale pipes, but it turns out that the flow is also sensitive to the size of the nanotube.
A closer look at the water flow in nanotubes made things even more complicated. In 2016 physicist Lydéric Bocquet of the Ecole Normale Supérieure in Paris and his co-workers carried out experiments showing that water flowing under pressure through carbon nanotubes gets faster as the tube diameter gets smaller than about 100 nm (Nature 537 210). In other words, nanotubes seem slippier the tinier they become. Yet for nanotubes made from boron nitride, the flow rates didn’t depend on tube diameter at all, which is just as one would expect from simple classical models.
Carbon nanotubes are made from concentric layers of graphene, which consists of carbon atoms arranged in a 1D honeycomb lattice. Graphene sheets are electrically conducting – they have mobile electrons – whereas boron nitride is insulating, despite also having a hexagonal lattice structure.
This difference made Bocquet and colleagues suspect that the unexpected behaviour might be somehow connected to the electron states in the tube walls. To add to the mystery, other experiments showed that water flows faster down nanoscale channels made of graphene than ones made of graphite – which is just stacked layers of graphene. The concentric layers of graphene in a carbon nanotube give them a graphite-like structure, so this could be key to understanding how water is transported through the nanotubes.
Resolving this tantalizing theoretical puzzle could have important implications for practical uses of nanotube membranes. “Such flows are at the centre of all sorts of processes in membrane science,” says Nikita Kavokine, a physicist at the Max Planck Institute for Polymer Research in Mainz, Germany. “We want to be able to make materials that perform better in terms of water permeability and ion selectivity.”
In 2022 Bocquet proposed a solution with chemist Marie-Laure Bocquet and Kavokine (who was then at the ENS) – the notion of quantum friction (Nature 602 84). They argued that water flowing over graphite can be slowed by a kind of drag created by the interaction of charge fluctuations in the water with wave-like excitations in the mobile electrons of the graphene sheets.
At first glance, it seems unlikely that very light electrons should interact with much heavier atoms and molecules, given that they move at such different speeds. “The naïve idea is that electrons move much faster than water molecules,” says Kavokine, “so they’ll never talk to each other dynamically.”
The big difference in timescales between the movements of electrons and atoms is after all the basis of the Born–Oppenheimer approximation, which lets us calculate the electronic states of atoms and molecules without having to worry about the effect of atomic motions. As Bocquet admits, when he and his co-workers first decided to explore the possibility of such an interaction, “we started with very vague ideas and not optimistically”.
But when the researchers did the calculations, they found that there was a way for the electrons in graphite and the molecules in water to feel one another. That’s because the thermal motions of water molecules create short-lived differences in density from place to place. And because water molecules are polar – they have an asymmetric distribution of electrical charge – these density fluctuations produce corresponding charge fluctuations called Debye modes within the liquid. The electron cloud in graphite also exhibits wave-like charge fluctuations, which behave as quasiparticles known as “plasmons” (figure 2).
According to statistical physicist Giancarlo Franzese of the University of Barcelona, the key to understanding quantum friction is to recognize that the properties of water must be treated as a many-body problem: the fluctuations that cause the Debye modes are collective, not simply the sum of single-molecule properties.
2 Gaining momentum
When water flows over a graphene or graphite surface, the electronic excitations called plasmons in the carbon lattice couple to the density fluctuations in the liquid, meaning that momentum and energy can be transferred between the two.
Bocquet and colleagues found that both plasmon waves in graphite and Debye modes in water may occur with frequencies of around several trillion per second – in the terahertz range. This means that there can be a resonance between the two, so that one can be excited by the other, just as singing a note loudly can set an undamped piano string vibrating if it has the same pitch.
In this way, water flowing over a graphite surface can transfer momentum to the plasmons within the graphite and thereby be slowed down, experiencing drag. In other words, the Born–Oppenheimer approximation breaks down here: an effect that Bocquet calls “a huge surprise”.
Crucially, the plasmons in graphite that couple most strongly to the water are caused by electrons jumping between the stacked graphene sheets. They don’t therefore occur in single sheets of graphene (figure 3). That, Bocquet and colleagues figured, would explain why water flows more slowly over graphite than over graphene – because only in the former case is there strong quantum friction.
3 Electron hopping
A schematic of the structure of graphite, and the interlayer plasmons that are associated with strong quantum friction. The “A” and “B” sublattices characterize the graphite structure, where “A” atoms sit directly between atoms in the neighbouring layers. The plasmon modes in graphite that couple most strongly to the charge fluctuations in water are caused by electrons jumping between the graphene sheets. Here the binding parameters describe the energy needed for electrons to tunnel between adjacent or second-nearest sheets.
But would it explain how the flow rate of water in a carbon nanotube depends on the tube diameter? In large nanotubes with diameters above about 100 nm, where the walls have relatively low curvature, the coupling of the electronic states between the stacked graphene layers is much the same as it is in normal graphite with flat sheets, so the quantum friction experienced by water flow is at its maximum strength.
But as the tubes get narrower and their walls become more strongly curved, the electronic interactions between the layers in their walls get weaker, and the layers behave more like independent graphene sheets. Below about 100 nm diameter the quantum friction declines, and if the tubes are narrower than about 20 nm there is none at all – the tubes are as slippery as the classical theories predict. So rather bizarrely, in this case, there seems to be less “quantumness” in the system as it gets smaller.
Rather bizarrely, in this case, there seems to be less “quantumness” in the system as it gets smaller
“Lydéric’s work is super-exciting,” says Angelos Michaelides, a theoretical chemist from the University of Cambridge in the UK, whose detailed computer simulations of the water–graphene interface confirmed that quantum friction occurs (Nano Lett. 23 580).
One of the strange characteristics of quantum friction is that, unlike its classical counterpart, it doesn’t rely on direct contact between the two substances in relative motion. Quantum friction would slow the water down even if there was a thin vacuum layer between it and the carbon nanotube. Sandra Troian from the California Institute of Technology in Pasadena, who studies the fluid mechanics of interfaces, says that this “friction at a distance” is related to a much earlier idea proposed in 1989 by the Russian physicist Leonid Levitov (EPL 8 499).
Fluctuations in the electron distribution around atoms means that neutral atoms, molecules and materials can exert a weak electrostatic force on each other called the Van der Waals force. Levitov argued that this could create a drag on the objects moving past each other, even when separated by a vacuum. “Levitov set the whole conceptual ball in motion by proposing that quantum effects acting at a distance can generate a frictional force without direct physical contact,” says Troian.
Plumbing the nanoscale
It all sounds good in theory, but could the idea be put to an experimental test? To do that, Kavokine has teamed up with Mischa Bonn, also in Mainz, an expert in using spectroscopy to probe the dynamics of water. At first, Bonn admits, he was sceptical. “I was like, guys, this is a really cool theory, but there’s no way you’ll see it at room temperature.” But he agreed to give it a try.
“Friction is momentum transfer,” explains Bonn. “But how can we measure that? Well, I can measure energy transfer – that’s what we typically do in spectroscopy.” So Kavokine rewrote the theory for quantum friction so that it quantified the energy transfer, rather than the momentum transfer. Then they set out to see if they could spot such energy transfer between the electron and water dynamics.
The calculations predicted that quantum friction is weaker in graphene than graphite, but Bonn’s team devised an experiment with graphene because they had already studied its electron dynamics. Bonn explains that the graphene monolayer has an in-plane plasmon that the water fluctuations can couple to, so quantum friction should still be present, though it will be a weaker effect than in graphite.
The researchers used optical laser pulses to excite the electrons in a single sheet of graphene immersed in water, in effect abruptly raising the “electronic temperature” so that it was out of equilibrium with the water (Nature Nanotechnol. 18 898). “There’s a certain intrinsic cooling time,” Bonn says – this is taken to be the cooling rate in a vacuum. “But if there’s significant energy transfer [between the graphene plasmons and the Debye modes of water] then that cooling rate should increase when there is water present.”
And that’s exactly what they saw. As the electrons cool, their ability to absorb light in the terahertz frequency range increases. By monitoring the absorption of terahertz pulses fired at different times after the initial exciting laser pulse, Bonn and colleagues could deduce the cooling rate. In this case, there seemed to be energy transfer between the water and the electrons – a signature of quantum friction – even for just a monolayer of graphene (figure 4).
4 Searching for quantum friction
A technique called “terahertz spectroscopy” was used to look for quantum friction. This technique measures the cooling rate of a material (in this case a sheet of graphene) after it is heated by a laser pulse. As the thermal excitation declines, the material’s ability to absorb radiation changes. By monitoring the absorption of a series of terahertz pulses, the cooling rate is calculated. Terahertz spectroscopy can be performed in a vacuum, or in a liquid bath. If the presence of a liquid causes the graphene to cool more quickly than in the vacuum, this indicates that there is quantum friction.
In contrast, when the graphene was immersed in methanol or ethanol, the cooling rate of the electrons was slower than in a vacuum. These are polar liquids but they don’t have Debye modes at the appropriate frequencies, and they merely inhibit the thermal relaxation of the electrons.
“My initial instincts were wrong,” Bonn admits merrily, “so it was a very pleasant surprise when it worked.” But while he says that the results are quantitatively consistent with the theoretical predictions, further experiments are needed to clinch it. What’s more, they have so far only looked at flat graphene sheets in contact with bulk water. “We really want to go to nanoconfined water,” he says – an extension they have already begun.
Beyond a pipe dream
Can quantum friction be put to good use? Kavokine hopes so, and has coined the term “quantum plumbing” to describe efforts to do so. “We can see how mechanical work [like fluid flow] can speak directly to electronic motion,” says Bocquet. “For example, if you move a liquid, you can induce an electronic current.”
The researchers are now thinking about how to exploit the direct conversion of energy between mechanical work and electron motion – for example, by harvesting the energy of waste flows to generate electronic currents, or using electronic control to alter flow rates and thus create nanoscale valves or pumps. “That is not impossible,” Bonn attests.
Kavokine points out that biological systems are – thanks to the fine structural tunability of proteins – very good at controlling flows on very small scales. While he thinks it “unlikely” that anyone could achieve that degree of structural tunability, “[our work] shows we can play instead with the electronic tunability to achieve similar functions with very different physics” – what he dubs an “anti-biomimetic route” to flow nanoengineering.
Understanding quantum friction could be useful for making low-friction materials, says Franzese. “Lubricants are often used as a solution, but many of them are not sustainable,” he says – so designing a material with intrinsically low friction would be a better option. What’s more, the approach of considering the nature of the water–solid interface as a many-body problem “could have implications in other fields such as filtering and separation of fluid mixtures”.
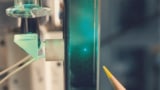
Cold: how physicists learned to manipulate and move particles with laser cooling
Meanwhile, Michaelides and Bocquet are exploring the idea of using the electronic excitations of a sheet of graphite as an intermediary to allow two flows on either side of it to communicate, such that one might induce the other: what they call flow tunnelling. Their simulations show that it should be possible in principle.
“I envision many important applications of this work [on quantum friction],” says Troian, “ranging from biological systems to those involving membrane-based separation, desalination, liquid batteries, nanomachines and more.”
Regardless of what quantum plumbers ultimately produce, as Bocquet neatly concludes, “it’s a very nice playground”.