Peer Fischer outlines the prospects for creating “nanoswimmers” that can be steered through the body to deliver drugs directly to their targets
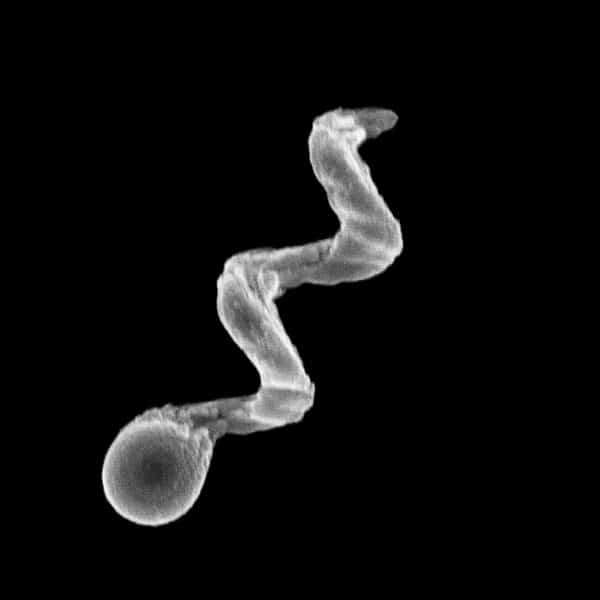
Molecules don’t move very fast on their own. If they had to rely solely on diffusion – a slow and inefficient process linked to the Brownian motion of small particles and molecules in solution – then a protein molecule, for instance, would take around three weeks to travel a single centimetre down a nerve fibre. This is why active transport mechanisms exist in cells and in the human body: without them, all the processes of life would happen at a pace that would make snails look speedy.
Active motion is also a property of many micro-organisms that swim to seek out food sources. Importantly, this process, which is known as chemotaxis, does not require the help of a central nervous system: although bacteria and other micro-organisms are alive, like the cells in our body, their swimming behaviour is entirely regulated by chemical feedback mechanisms. This means that active transport is not necessarily restricted to living matter. Indeed, many have wondered whether it could be used to propel synthetic nanoparticles as well – if, in short, one could make nanoparticles that actively “swim” through their environment in a way that can be controlled, or that responds to external signals.
Realizing such a “swimming nanoparticle” scenario would have far-reaching implications for nanomedicine. Directed nanoparticle transport would, for example, allow clinicians to deliver a pharmaceutical compound to a small “target” region where it is needed, rather than to the entire body. Such controlled delivery would avoid unnecessary systemic exposure to toxic chemicals and might even allow the use of more potent drugs. The controlled delivery of nanoparticles could also greatly enhance existing nano-based cancer treatments. For example, nanoparticles sometimes accumulate in tumours on their own, due to differences in the vasculature of tumours compared with the blood vessels that supply nutrients to healthy tissues. If these nanoparticles are made from materials that absorb radiation, or respond to A/C magnetic fields, then the nanoparticles can be heated, causing tumour tissue to die of hyperthermia. However, so far, experiments on this type of treatment have relied on passive transport, meaning that the nanoparticles are not controlled or well localized in the tumour.
Magnetic motion
Here, then, is the critical question: how can one actively direct nanoparticles to a specific location in the body, such as a tumour? It will come as no surprise to physicists that a force is required to direct and concentrate nanoparticles in a region of interest. For instance, magnetic particles can be pulled in a magnetic field gradient, while even non-magnetic ones will experience a force in focused light beams or chemical gradients. However, establishing sufficiently strong gradients across a large volume, as would be required inside the body, is challenging. Finding means of propelling particles that do not require the application of external gradients is therefore an active area of research, and some progress has already been made. It is possible, for example, to actively move micro- and in some cases nanoparticles with a gradient-free magnetic or ultrasound field, or with chemical reactions.
Another difficulty is that controlling the motion of particles inside the body is far from trivial, especially if the particles need to penetrate into tissues rather than simply moving through the bloodstream. Both tissues and fluids (such as the mucus that lines our lungs, stomach and intestines) serve as a barrier to keep bacteria and other pathogens out. Although biomedically important fluids and tissues largely consist of water, they also contain dense networks of macromolecules that could impede the passage of nanoswimmers. For example, the fluid between the lens and retina in your eye – the vitreous humour – consists of a network of collagen fibrils, proteins and long-chained polysaccharides with a mesh size of a few hundred nanometers, which is difficult for larger particles to penetrate.
Current research studies how self-propelling particles move, interact and give rise to self-organization and collective behaviour
To address this challenge, my research group has developed fabrication methods that enable us to grow hundreds of billions of magnetic particles in the shape of corkscrew propellers. These nanopropellers (see image) can be grown from a range of materials, including biocompatible ones such as silica, gold or iron oxide, and their diameters range from 40 nm to a micron. They possess a magnetic moment orthogonal to their long axis and when a rotating homogenous magnetic field is applied, the screw-shaped particles begin to rotate as well, moving forward like tiny drills. If we make the nanopropellers small enough, they can slip through networks of macromolecules. In effect, the nanodrills are able to meander between the macromolecules and fibrous structures that impede or prevent the transport of larger particles. They also easily propel themselves through the water that fills the interstitial space (2014 ACS Nano 8 8794).
Decorating enzymes
An alternative strategy for helping nanoparticles move through viscous fluids is to “decorate” them with enzymes that facilitate their transport. This option mimics the strategies used by certain pathogens to move through environments within the body that would otherwise be hostile to their progress. For instance, the bacterium Helicobacter pylori has developed a strategy to swim through the mucus that lines the walls of the stomach. Its trick is to excrete urease enzymes, which locally increases the pH via a chemical reaction and thus causes the mucus to become much less viscous and easier to penetrate. Anchoring urease enzymes to our nanoparticles thus enables their transport through mucus (2015 Sci Adv. 1: e1500501).
The appeal of developing particle-based agents is that they can be made from different materials, and can thus be equipped with a range of properties. For non-magnetic particles, it is possible to obtain active motion purely from chemical reactions. A particle can, for example, propel itself if it is partially covered with a catalyst and immersed in a fluid containing molecules that react in the presence of the catalyst. Such chemically active particles show, in addition to their Brownian random walk, an active component to their motion. Because the distribution of the catalyst over these particles is asymmetric, the product molecules that form during the chemical reaction will also be unevenly distributed across the particle. This results in a local chemical gradient, which, in turn, causes fluid to flow near the particle surface and gives rise to the “self-propulsion” of the particle. Such actively moving particles are also known as chemical motors and they have been proposed as potential drug carries for medical applications, especially if the reaction that propels them is one that can feed off molecules such as glucose. Current research therefore studies how self-propelling particles move, interact and give rise to self-organization and collective behaviour. The latter arises due to interactions of the active particles in the fluid, and may include phenomena such as clustering, macroscopic flows and sometimes even the formation of particle swarms.
While many self-propelled particles are fuelled by toxic chemicals, such as hydrogen peroxide, it is also possible to use enzymatic reactions to produce active motion. As the “engines” get stronger and the chemistry more sophisticated, it even becomes possible to cloak particles. Coating particles with the membranes of blood cells, for example, effectively shields them from the body’s defence mechanisms.
Regardless of whether nanoswimmers are externally driven or chemically powered, micro- and nanoparticles still need to overcome a number of hurdles before they will be fit for clinical drug delivery. Controlling their motion is only one aspect of the problem: more generally, one also needs to consider the biocompatibility of materials, and it may be necessary to consider how the particles could be removed after they are applied. However, in some special cases, the need for new treatments could outweigh some of the potential risks. For instance, solutions are sought for the treatment of aggressive brain tumours, where long-term effects are not a first concern, and it could be here that such specialized active treatments can make their mark. However, both the treatment of tumours or even microsurgery with guided nanoswimmers will require more research to develop the necessary science and to advance this fascinating field of nanotechnology.