Molecules with over 100 atoms can be made to interfere, according to recent experiments that study the transition from the quantum to the classical world. Markus Arndt, Anton Zeilinger and Klaus Hornberger explain
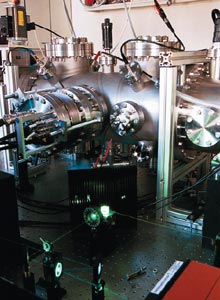
Ever since quantum theory was developed during the first quarter of the 20th century, we have lived with a strange division. Objects in our daily lives behave “normally” – they appear to obey classical physics – whereas microscopic objects can behave counterintuitively and reveal intriguing features of quantum physics. But where exactly is the boundary between the quantum and classical worlds – if, indeed, there is one? If quantum physics is a universal theory, why is it respectable to talk about the quantum behaviour of electrons but not, say, of footballs?
One way of answering these questions is to carry out sensitive interferometry experiments, in which a beam of molecules is sent down two different paths and then brought back together again. These experiments reveal that the molecules have both a “wave” and “particle” nature, and display quantum behaviour. Recent research by our group has shown, in fact, that molecules with as many as 100 atoms can interfere with one another. These experiments illustrate one of the most unusual aspects of quantum theory, namely that objects can exist in a superposition of different states.
This “non-locality” is often loosely described as “a single object being in two different places at the same time”. However, this casual description is meaningless. To see why, consider the familiar Young’s double-slit experiment, which still forms the basis of many modern investigations in quantum optics (figure 1a). In its simplest form, light from a bright lamp covered by a coloured-glass filter passes through a single slit and then through two other slits, which are positioned very close together, before landing on a screen.
If the slits are sufficiently narrow and the wavelets of light that emerge from them are coherent – i.e. have a well-defined phase relationship over an extended region of space and time – then a pattern of bright and dark fringes will be produced on the screen. These fringes correspond to areas where the wavelets have interfered constructively and destructively, respectively. To verify that interference has taken place, we need simply close one of the double slits, which will make the pattern disappear.
But as Geoffrey Ingram Taylor first demonstrated in 1909, if you dilute the source of light so that there is just one photon in the apparatus at a time, you will still observe a full interference pattern, provided that you wait long enough. So what exactly is interfering in this case? As we need at least two wavelets to generate an interference pattern, could it be that a single photon is going through both slits at once?
Questions without answers
Unfortunately, these questions are impossible to answer, even in principle, because we cannot have both perfect interference fringes and full information about which path the photon has taken; we can only have one or the other. If both slits are open, we obtain interference, but we then have no information about which slit the photon went through. Conversely, closing one of the slits singles out the path information, but completely destroys the interference pattern. This is a simple example of what Niels Bohr called the complementarity principle. (Note that if we have partial path information, i.e. if we can tell the paths apart with a certain probability, we could obtain interference, although the contrast between the fringes would not be as great.)
We cannot therefore assign any reality to the photon’s position as it travels through the interferometer because we have no way of verifying its path (i.e. its particle nature) without affecting the interference pattern (i.e. its wave nature). The only thing we know is whether a photon has reached the detector and, if so, that there is a well-determined probability that it passed through either one of the slits. These observations may seem strange, but things become even more counterintuitive when we consider interferometry with massive particles, such as molecules.
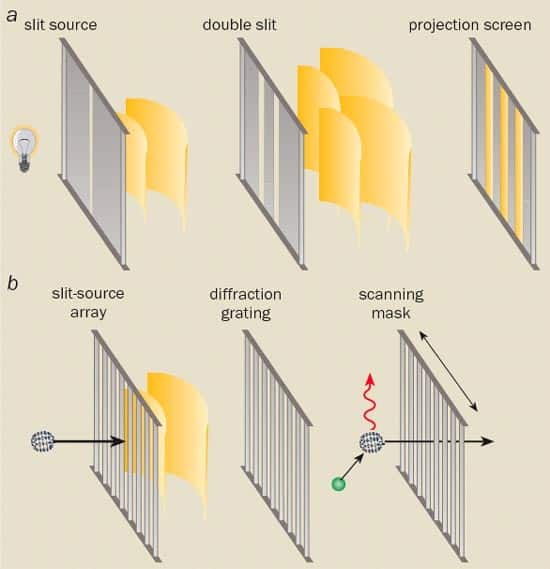
Can molecules be in two places at once – i.e. “delocalized” – and show wave interference? And what about even larger objects, like footballs: could they interfere? In principle they could, because any particle can be associated with a wave that has a de Broglie wavelength λ = h/mv, where h is Planck’s constant, m is the mass of the particle and v is its velocity. Unfortunately, the de Broglie wavelength of something as big as a football will be vanishingly small and render the experiment impossible because the fringes would be too close together.
And while it is possible to observe interference with smaller objects like electrons, atoms and molecules, we have to remember that they can interact with their surrounding environment by colliding with other molecules or by exchanging electromagnetic radiation. The environmental state gets “entangled” with the quantum object, which means that information about the whereabouts of the object is rapidly disseminated into the surroundings. As a result, the two systems are correlated even after the interaction has taken place. And since we could, in principle, obtain that information – even if an observer never actually does so – the interference pattern vanishes.
This loss of coherence – or what we call “decoherence” – is one of the crucial reasons why macroscopic objects in our daily lives never show quantum phenomena, such as interference. They are so big that they interact strongly with the environment and lose their coherence very quickly. In other words, the absence of quantum behaviour in the macroworld arises naturally because it becomes increasingly difficult to isolate objects of increasing size and complexity. Remarkably, it is the quantum nature of the interaction with the environment and the resulting information transfer that leads to the classical behaviour of a quantum object.
Although Heisenberg, Einstein, Bohr and many of the other founding fathers of quantum theory had thought about whether interference could be destroyed by knowing the path information of a quantum object, for a long time it seemed a rather abstract, philosophical question (see box). Only recently have experimental advances made it possible to study the effect of an external influence on the interference of atoms and molecules. These experiments are not just of fundamental importance for the understanding of our classical world. They are also relevant to quantum computers – devices that could, in principle, out-perform a classical computer by exploiting the quantum-superposition principle. Decoherence is thought to be the main stumbling block in turning a quantum computer from theoretical dream to practical reality.
Interferometers: where matter waves matter
In recent years several research groups have managed to carry out “matter-wave” interference experiments with a wide range of objects from electrons to large molecules like buckyballs and beyond. However, it is not easy to use conventional double-slit experiments to study the interference of such particles. The problem is that very massive particles have extremely small wavelengths. The slits would therefore have to be tiny and the beams very tightly collimated in order to obtain diffraction using a double slit or grating.
Despite the experimental difficulties, Olivier Carnal and Jürgen Mlynek from the University of Konstanz in Germany did manage to observe interference in a double-slit experiment with atoms in 1991. While around the same time, David Pritchard and co-workers at the Massachusetts Institute of Technology (MIT) showed that a wider range of experiments could be carried out using a different device known as a “Mach-Zehnder interferometer”. In their device, a beam of atoms passes through an array of vertical slits, where it is split into at least two different coherent wavefronts separated by as much as 17 μm. Both wavefronts then pass through a second, identical array that brings them back together again. The interference creates a periodic rise and fall in the density of atoms in a certain plane behind the second grating. This is measured by a third grating that is designed such that the gap between the slits is the same as the expected period of the interference pattern. By moving the grating perpendicularly to the beam, a steady rise and fall in the rate of transmitted atoms can be observed.
Using this device, Pritchard’s team successfully obtained interference fringes with sodium atoms – clear proof of the delocalization of atoms in free flight. So what happens if you probe a single atom as it travels along one of the two possible paths before undergoing interference? Theory suggests that this should wipe out the interference pattern.
In 1995 Pritchard and co-workers investigated this question by directing a laser at the atom beam as it travelled between the first and the second grating. Whenever they positioned the laser such that the beam path could be optically resolved from any photon scattering off the beam, the atomic interference pattern did indeed disappear – just as Bohr’s complementarity principle says it should. Provided that the wavelength of the photon is less than twice the distance between the separated atomic wavefronts, it will carry sufficient “which path” information to destroy the interference pattern.
Going big: molecule interferometry
Matter-wave interference experiments have progressed in leaps and bounds in recent years. But what are the experimental, technological and physical limits of matter delocalization? Do the mass, temperature and complexity of the particles have any bearing on whether they interfere? Will particles that are geometrically asymmetric or have a permanent electric dipole moment interact more strongly with the environment and so become decoherent more quickly? Questions like these inspired us to start experiments on molecule interferometry at the University of Innsbruck in 1998. These continued when our group moved to Vienna in 1999, where we are still based.
In a first, proof-of-principle experiment, we decided to observe the quantum-wave nature of fullerene molecules undergoing grating diffraction. Fullerenes are a class of closed-shell carbon molecules, the most common consisting of 60 carbon atoms arranged like a soccer ball with a diameter of 1 nm. These objects are, in many respects, classical bodies, particularly because they can store a lot of internal energy in many degrees of freedom. For example, when heated to about 3000 K, fullerenes can emit electrons, photons and even diatomic carbon molecules – just as a little block of hot solid material glows, emits black-body radiation and cools through evaporation.
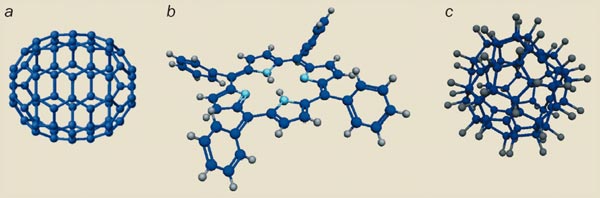
Molecular-interference experiments are far from easy. We use commercially available fullerenes that we heat to about 900 K to generate an intense molecular beam. (They would disintegrate if we heated them to higher temperatures for a long time.) Most of the molecules are then travelling at about 200 m s-1, which means that they have a de Broglie wavelength of just 2.5 x 10-12 m – some 400 times smaller than the size of the molecule itself. We therefore need extremely narrow gratings so that the molecules leave the grating at a large enough angle.
Luckily for us, Tim Savas and colleagues at MIT developed gratings with a slit separation of 100 nm. In 1999 we also managed to design a very sensitive detector that can locate the positions of individual fullerene molecules. It uses a tightly focused intense laser beam that ionizes the molecules; scanning the position of the focal point of the laser transversely across the molecular beam reveals high-contrast interference fringes.
To extend these experiments to more complex and more massive particles, we built a variant of the Mach-Zehnder device, known as a Talbot-Lau interferometer. It has several advantages. First, the individual gratings are positioned much closer together, which makes the interferometer shorter and more rugged. Second, we can use a spatially incoherent beam containing a mixture of plane waves incident from various directions. The beam does not therefore have to be precisely collimated, which means we can use many more particles and significantly increase the signal-to-noise ratio.
Another big advantage of the Talbot-Lau interferometer is linked to the fact that the de Broglie wavelength of a particle is inversely proportional to its mass. To detect interference of more massive particles you therefore have to improve the resolution of the detector and have slits that are closer together. For a simple diffraction grating, this means that the grating constant, i.e. the grating period or separation between the slits, has to be decreased by the same factor that the mass is increased. But with near-field Talbot-Lau interferometry, the grating constant only has to be decreased by the square root of the particle mass, as John Clauser, who was then at the University of California at Berkeley, first pointed out in the mid-1990s. The technique therefore offers good spatial resolution and works even with massive particles that have a very small de Broglie wavelength. In practice, for the same molecular beam we needed a grating period of 100 nm for far-field diffraction and a period of only 1000 nm for near-field interferometry, which is much easier to fabricate and operate.
To prove that the molecules interfere, we use a third grating that has the same period as that of the expected interference pattern. By scanning the position of the grating, we ought to see an alternating rise and fall in the count rate of transmitted molecules. The quality of these almost sinusoidal fringes can then be quantified in terms of the contrast between the light and dark bands of the interference pattern. We have achieved typical contrasts of 40-50% for carbon-70 buckyballs, which agrees with quantum theory if we include all the experimentally relevant parameters.
In 2003 we used the same set-up to prove the wave nature of even bigger molecules, such as the biomolecule tetraphenylporphyrin (C44H30N4 or “TPP”) and the fluorinated buckyball C60F48 (figure 2). The pancake-shaped porphyrins were particularly interesting because some physicists had argued that only molecules that are highly symmetric or even spherical would interfere. However, C44H30N4 – a derivative of a biodye that is present in chlorophyll – is over 2 nm wide, and thus twice as broad as the football-shaped carbon-60 molecule. Quite clearly, the shape of a molecule does not affect its interference properties at this scale. As for the fluorinated buckyball C60F48, it currently holds the world record for the most massive single particle to display quantum interference. Although it is not as extended as the porphyrin, it has an average atomic mass of 1632 units and contains 108 atoms covalently bound in a single interfering object.
Decoherence in a molecule interferometer
These experiments show us that even large and complex molecules can interfere and reveal their quantum nature. But molecules are usually seen as well-localized objects that we can even observe using high-resolution microscopy. So what are the effects destroying the molecule’s delocalization and wiping out the fringe pattern? In fact, there are at least two relevant mechanisms that make it possible to measure the position of a molecule. The first involves collisions with other particles, such as gas molecules, while the second involves thermal radiation emitted by the molecule.
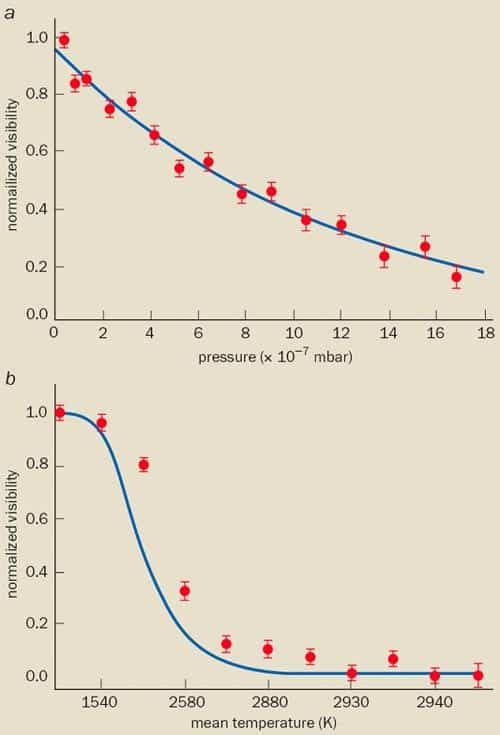
To find out how these processes can destroy the interference pattern and lead to classical behaviour, we gradually added gas to the chamber of our Talbot-Lau interferometer during the experiments with carbon-70 molecules (figure 3a). We found that the amount of contrast between the interference fringes fell exponentially as more gas was added, and that the fringes disappeared almost entirely when the pressure had reached just 10-6 mbar. This was in full quantitative agreement with a theoretical analysis of the scattering processes. Although a single collision with a gas molecule will not kick the massive fullerene out of the interferometer path, it is enough to destroy the interference pattern because it carries sufficient information to determine the path that the interfering molecule has taken. The exponential decay is thus directly related to the collision probability. Calculations suggest that molecules could have an atomic mass of as much as one million and still be unaffected by collisional decoherence in a realistic Talbot-Lau interferometer at a pressure of 10-10 mbar. Such pressures are perfectly feasible with existing vacuum technologies.
We then looked at how a molecule’s “internal temperature” affects interference. The concept of internal temperature is not relevant for atoms or electrons, but it is for molecules, which are complex objects. It describes the energy distribution of the many vibrational and rotational degrees of freedom. Hot objects, of course, emit thermal photons that are then absorbed by the environment, transferring momentum in the process. In other words, each photon can transfer information about the position of the emitting object that can, in principle, be measured. Indeed, when we increased the internal temperature of carbon-70 molecules to above 1000 K, the contrast between the interference fringes slowly disappeared (figure 3b).
We have also developed a theoretical model that can explain these observed decoherence rates. Based on an adapted version of Planck’s law, it describes how increasing numbers of short-wavelength photons are emitted as the molecule’s internal temperature increases. All it takes to destroy the interference fringes is for the molecule to emit either lots of long-wavelength photons or a single photon with a wavelength shorter than twice the separation between the coherently split molecular wavelets. This separation, which is the distance between two neighbouring grating slits, is 1 μm in our near-field set-up. The good agreement between the predicted and the measured decoherence rate indicates that the carbon-70 molecules emitted a few visible photons (~400-800 nm) when they were heated to internal temperatures above 2500 K.
This experiment proves three things. First, it shows that decoherence due to heat radiation can be quantitatively traced and understood. Second, it confirms the view that decoherence is caused by the flow of information into the environment. In matter-wave interferometers, which only observe the centre-of-mass motion alone, information can only be mediated by a transfer of momentum. Finally, it shows that thermal decoherence is relevant for truly macroscopic objects. Fortunately, it will be less of a concern in future interferometry experiments with large molecules, clusters or nanocrystals. Objects like these will have to be substantially cooled to make them coherent and to suppress the emission of thermal radiation.
Which-path information without momentum transfer
Another way of studying decoherence is to encode the interferometer “path” information in an external system. For example, Serge Haroche and colleagues at the Ecole Normale Supérieure in Paris used a beam of excited rubidium atoms, in which a laser had promoted the outer electron to a very high energy level to create a “Rydberg atom”. However, the experiment did not involve sending the atoms down different branches of an interferometer. Rather, the researchers observed the evolution of different internal states.
In this “Ramsey interferometer”, a pulse of microwave radiation creates a coherent superposition of an individual Rydberg atom in its ground and excited states. A second microwave field, located further along the beam, then recombines the different states to create interference fringes in the population of the ground state when the effective pathlength was varied inside the interferometer. Haroche and colleagues were able to extract information about the internal state of the atoms by placing a microwave resonator between the two microwave pulses. Since the phase of the microwave field changes when it interacts with an atom, information about the atoms passing through the resonator becomes encoded in the cavity field. This entangles the atomic and the field state without significant momentum transfer.
When the cavity was empty – so that no which-path information could be deposited – the Paris team saw high-contrast atomic interference fringes. But when a small coherent field, containing as few as nine photons on average, was added to the resonator, the fringes became less pronounced. This indicated that the interference had been destroyed due to the entanglement with the phase of the mesoscopic coherent photon field.
Various other researchers have also explored the possibility of encoding position information in the internal states of the interfering particle itself. Back in 1987, for example, Helmut Rauch and colleagues in Vienna used a Mach-Zehnder interferometer with polarized neutrons and encoded the path of the particle using its spin. Gerhard Rempe and co-workers at the Max Planck Institute for Quantum Optics in Garching, meanwhile, recently used two different hyperfine ground states of rubidium to mark the path in atom interferometry. Both experiments confirm the view that matter-wave interference vanishes if the two different position states of the interfering object are correlated with orthogonal internal states.
Entanglement between a quantum particle and its environment is not the only way of destroying interference. Noise – due to our inability to control all the experimental conditions sufficiently well – can also be a problem. In particular, experimentalists will have to fight the fact that random fluctuations in the relative length difference between the two interferometer arms can tend to wash out the interference pattern. Moreover, as the molecules get bigger and the de Broglie wavelength shrinks, experiments become increasingly sensitive to these effects. In our current molecular interferometer, the relative arm length is stable to better than one-thousandth of the diameter of the molecules. Although the experimental requirements will become even more demanding in the future, we are optimistic that these barriers can be overcome.
Decoherence and the quantum-classical boundary
Ever since the dawn of quantum theory, people have been struggling to reconcile the weirdness of its rules with our everyday experience. If it is perfectly permissible for a quantum particle to be at “different places in the same instant of time”, why then do all the tangible, macroscopic objects that we can see and feel obey classical physics?
The early generations of quantum physicists, led by Niels Bohr, Werner Heisenberg and John von Neumann, insisted that there is a strong division between the classical world and the quantum realm, although they conceded that the boundary is not fixed by the laws of physics. Their view was that the transformation into “classicality” is effected by the very act of observation; the idea being that the wavefunction “collapses” to a particular value when the observation takes place. To avoid the seemingly decisive role played by the observer, physicists put forward many alternative theories and interpretations. Often this was done at the price of introducing as-yet-unobserved quantities into quantum mechanics called hidden variables.
Decoherence theory, in contrast, is based firmly on the conventional framework of quantum mechanics. One avoids the question of “when does a collapse occur?” by arguing that all macroscopic objects – including the measurement apparatus – are ruled by the Schrödinger equation. Decoherence cannot therefore solve the philosophical problem of understanding the human perception of a particular reality. However, it can explain the emergence of classicality, that is how and when an object loses its quantum features and becomes indistinguishable from a classical description.
The crucial point is to acknowledge that no quantum object is completely isolated; rather it is embedded in an environment consisting of gas particles, photons and the like. The environmental state gets easily “entangled” with the quantum object, which causes information about the whereabouts of the quantum object to be rapidly disseminated into the surroundings. The absence of quantum behaviour in the macroworld is a natural consequence of the fact that bigger and more complex objects are much harder to isolate. In other words, the quantum features of the environmental interaction and the resulting information transfer lead to the appearance of classicality in quantum objects.
Why it all matters
Matter-wave experiments show that there is no fixed boundary between the classical and quantum worlds. An object can behave quantum mechanically in one experimental setting and classically in others. What seems to be the key factor in the transition from quantum to classical behaviour is the exchange of information between the quantum system and the outside world. This transition only depends on whether the experimental set-up allows – or does not allow – such information about the quantum system to be revealed. In the case of interference experiments, all that matters is that “which path” information is, in principle, available to the outside world. Contrary to popular belief, it is irrelevant whether a person actually makes the effort to find out that information.
In our view, matter-wave interferometry should be feasible for large objects such as proteins, small viruses and nanocrystals with atomic masses of up to 106 units. Extrapolating our results to bigger masses and higher temperatures, we believe that neither collisions nor thermal decoherence will be a problem in these cases. No fundamental limit of quantum interference is yet in sight, but much work still has to be done to prepare and manipulate coherent beams of supermassive particles. Carrying out such experiments will be a fascinating challenge.