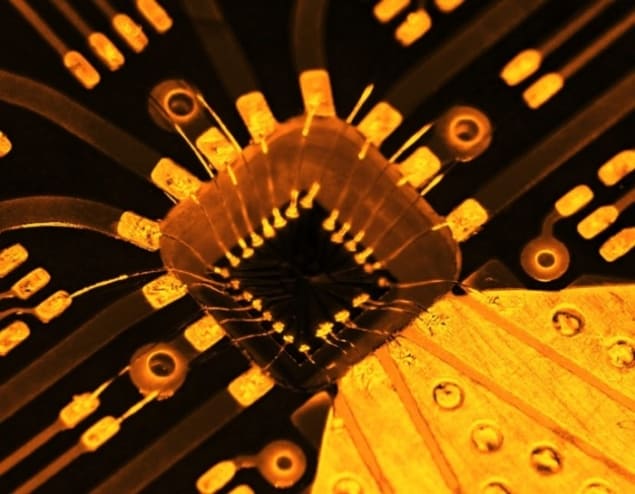
An important threshold in quantum error correction has been reached using silicon-based qubits. The feat was performed by three independent research groups, who used the spins of individual electrons or nuclei to create quantum logic gates, which carried out operations with over 99% fidelity. Silicon is widely used in the microelectronics industry, so this development could lead to quantum computers based on the large-scale integration of silicon devices.
One team was a collaboration between researchers at the Japan’s RIKEN Centre for Emergent Matter Science and QuTech in the Netherlands. The second was an international team led by researchers at the University of New South Wales (UNSW) in Australia and the third team was based solely at QuTech.
Today’s nascent quantum computers are delicate devices that are constrained by the fact that their qubits can only maintain superpositions of multiple quantum states for a limited amount of time. Beyond this “coherence time”, qubits can lose their quantum information, introducing errors into calculations. To get around this problem, physicists are developing error correction algorithms to keep calculations on track. However, error corrections schemes require qubits that have at least a 99% fidelity – meaning that they only fail 1% or less of the time.
Such high-fidelity operations have been achieved in qubits made of superconducting circuits, trapped ions, and nitrogen-vacancy centres in diamond. However, these qubits are relatively large, which limits their practically when it comes to integrating large numbers of qubits in a single device.
Material of choice
Silicon is the material of choice when it comes to large-scale integration, and physicists have been developing qubits based on that material – often using the spins of individual electrons as qubits. To become competitive with existing approaches, operations involving these qubits would need to clear the 99% fidelity threshold, while ensuring that the spins are both accessible and easily controllable.
The Riken–QuTech and QuTech teams met these requirements by fabricating two nearby quantum dots within a silicon chip. The quantum dots behave much like atoms, each trapping and isolating an electron. Electric fields created by electrodes on the chip were then used to control the qubits.
These two teams used their qubits to implement quantum algorithms, which involved configuring the qubits to create single- and two-qubit logic gates. To do this, the researchers applied an electric field to act as a barrier between pairs of quantum dots. When this barrier is lowered, both electrons moved closer together until their wavefunctions overlapped – causing their quantum states to become entangled.
Implanted ions
The UNSW-led team took a different approach in creating its qubits. The researchers began with a silicon chip into which they implanted two phosphorus ions nearby to each other. Each ion has a nuclear spin, allowing them to be used as qubits. These ions are very well isolated within the silicon, so the qubits have long coherence times. The two phosphorus qubits are coupled using a nearby electron that is manipulated in a single-electron transistor on the chip. Microwave pulses are used to control the process.
In all three studies, quantum gate fidelities exceeding 99.5% were achieved, which is well above the widely accepted threshold for error correction. Each team took a different approach to demonstrate this advantage. The Riken–QuTech team implemented two quantum error correction algorithms. Here, the algorithms each achieved success rates as high as 97%, showing that silicon-based quantum computers can realistically perform highly accurate calculations.
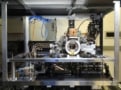
Physicists achieve fault-tolerant control of an error-corrected qubit
To demonstrate the capabilities of its qubits, the QuTech team successfully calculated the dissociation energy of molecular hydrogen from first principles – which is a highly demanding task when done on a conventional computer. Finally, the UNSW-led team demonstrated entanglement between its two phosphorus qubits and the intermediary electron, thereby creating a three-qubit state. Since the electron spins in semiconductors can be further coupled to other electrons, or physically shuttled to other locations, this achievement presents realistic routes towards scalable quantum information processing, using a combination of nuclear and electron spins.
The results of all three studies now bring a silicon-based quantum computing platform a step closer to reality. However, much more work needs be done and the teams now aim to achieve larger arrays of two-qubit gates that remain above the 99% error correction threshold. If successful, large-scale quantum computers based on silicon may one day become a reality.