Biophysicists are now able to study a whole host of living processes with unprecedented accuracy thanks to a microscope normally associated with surface science
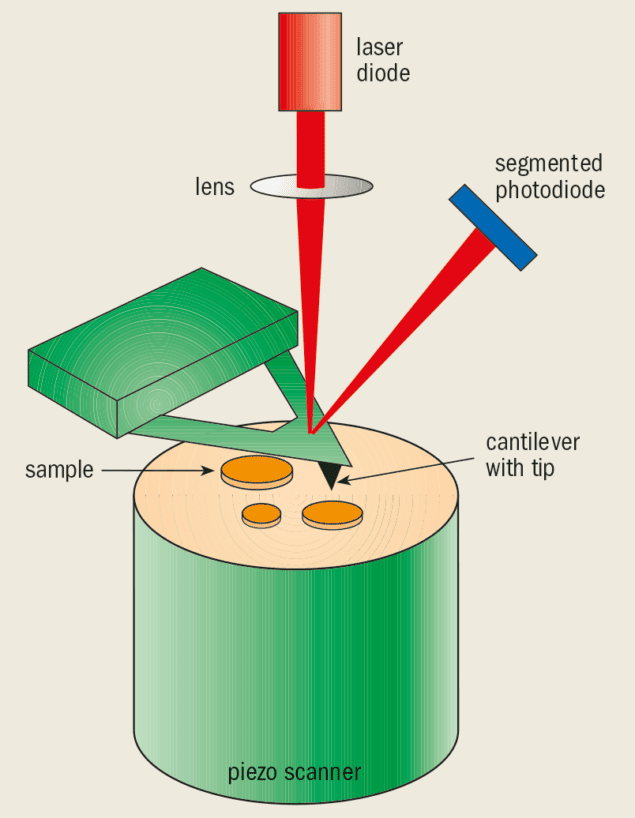
Biological cells, molecules and organisms are complex systems that are increasingly capturing the imagination of physicists. Unlike many objects in the physical domain, biological systems cannot simply be described by the collective behaviour of individual components. The difference is that biological cells are living systems – a property that has less to do with the number of components they contain and more to do with the way these various components are arranged. Many physicists recognize that understanding this complexity is a fundamental challenge of biology.
Yet the components of a living cell obey the same laws of physics as all other systems, from stars and galaxies down to the smallest subatomic particle. Although there are four fundamental forces in nature, the electrostatic force is the most important in biology. (We will overlook some special cases where the gravitational force also plays an important role, such as the stability of trees and the flow of blood in a giraffe’s neck.)
As an example of the way in which biological systems are governed by the laws of physics, consider the concept of “charge screening” in an ionic solution. The electric field around a positively charged ion, for example, will effectively decrease with distance because negatively charged ions cluster around it. Charge screening is important in a whole host of physical systems, from plasma physics to colloids. Although most biological molecules and membranes are charged, the charges only play a role at very small distances.
Unlike chemical reactions in a test tube, the reactions in a biological system cannot simply be started or speeded up by changing the temperature. In biological systems, enzymes are used as catalysts to start and control reactions because the temperature is either fixed by the environment or by the organism itself, as in the case of warm-blooded animals.
Despite their complexity much can be learnt about complex biological systems by studying their individual components. And in recent years new experimental techniques have been developed that allow researchers to study and manipulate individual molecules. These range from single-molecule fluorescence and optical tweezers, where laser beams are used to control biological specimens (see Padgett and Allen in further reading), to scanning-probe techniques, such as atomic force microscopy.
Versatile device
Atomic force microscopes (AFMs) are particularly well suited to studying biological systems thanks to their versatility. These instruments can be operated in many different ways, ranging from simply mapping the surface of a sample in detail to measuring the local forces on its surface. Another advantage of the AFM is that we can also study the properties of living molecules as they undergo various reactions, and thereby learn how they function.
An AFM comprises a very sharp tip, typically 10-10 m in radius, attached to a weak cantilever spring that can be brought into direct, mechanical contact with the sample (figure 1). The sample is typically mounted on a piezoelectric “positioner” that can be moved in all three dimensions. We can obtain a contour map of the surface of the sample by simply moving the tip over the surface in two dimensions and measuring the way the spring bends. Or by pressing the tip into the sample, we can apply a force to a specific position on the sample. By pulling the tip away from the sample we can also measure the force between the two to an accuracy of around 10 pN. Thanks to this high sensitivity, the AFM can probe and study the structure and function of single, intact biological molecules.
Elasticity of single molecules
How far can a polymer molecule stretch? This is one of the most important factors in determining how certain molecules function. For instance, some antibodies have to uncurl and stretch in order to fight disease-producing organisms. And the elasticity of connective tissues, such as collagen, is clearly important.
In 1997 Mathias Rief and co-workers at the University of Munich used an AFM to measure the elastic properties of a single dextran molecule – a long sugar molecule that is produced by certain bacteria. The basic idea is to hold one end of the molecule tightly by binding it to a substrate and to pull the other end with the tip of the AFM. The distance between the tip and the substrate is then increased steadily. As the dextran molecule is pulled, it changes shape and the force is measured by monitoring the deflection of the cantilever spring. The variation of the force with this distance is known as a force-extension curve (figure 2).
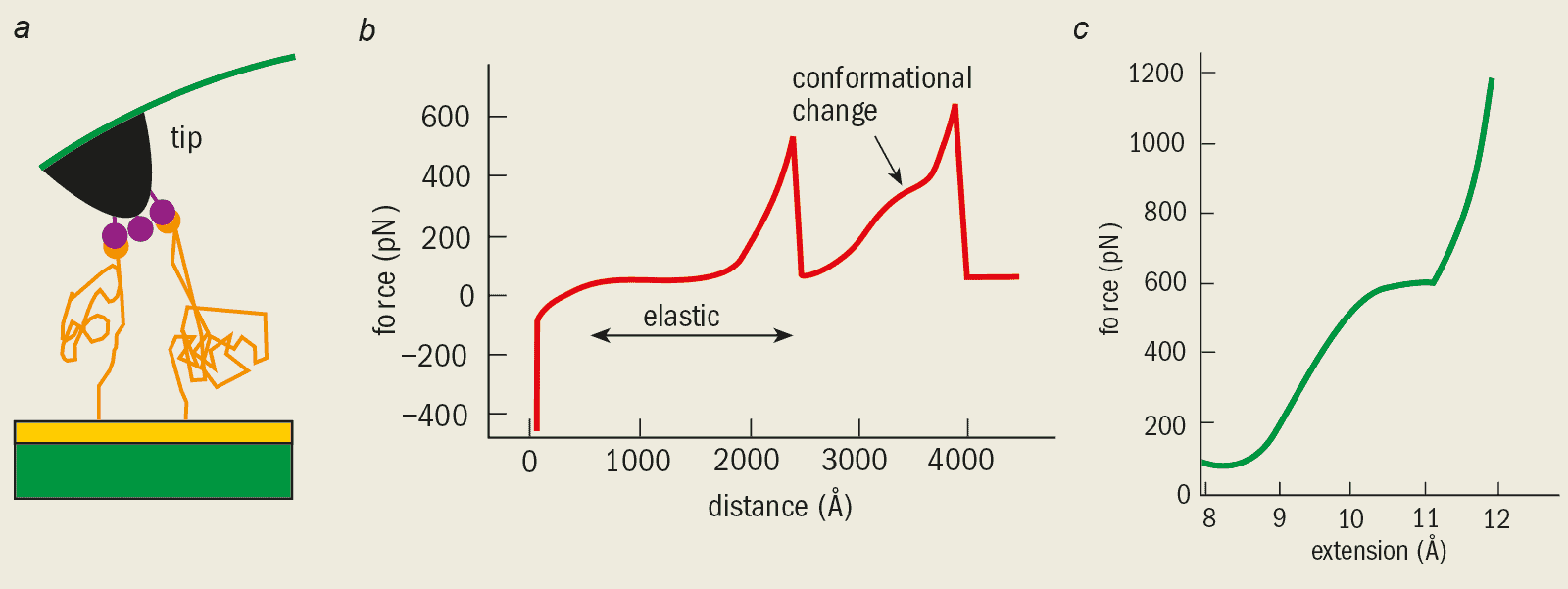
The force-extension curves from such experiments can be understood by comparing them with various models based on polymer theory. The dextran molecule has a very flexible structure and when small forces are applied its elastic properties are well described by the so-called “rubber elasticity model”. In this model, the polymer chain is thought of as a series of smaller compounds called monomers that are linked by rotating joints. As no energy is needed to deform these joints, the polymer can easily bend into any shape or conformation. Often this type of conformation is called a random coil.
However, molecules are likely to bend into some shapes or “conformations” more than others. The biological and chemical behaviour of the molecule is entirely governed by the number of different shapes it can form. When we pull a molecule with an AFM, we change the number of possible shapes that it can adopt, and an energy price has to be paid that changes with the temperature.
We can describe this behaviour more easily by considering a rubber band that has been stretched, for example, by suspending a small mass from it. If we heat the band, it will shorten and become stiffer. As a molecule is stretched further, an additional elastic force is measured and eventually the stiffness of the molecule changes. It is clear that rubber bands and molecules behave very differently from stretched springs.
These different regimes can be fully understood if we look at the process of pulling and deforming the molecule in atomic detail, using simulations of the molecular dynamics. In these simulations, the motion of each atom is calculated due to the forces it experiences from the other atoms. The simulations can only be performed numerically with the help of computers and are very time consuming. Even the fastest computers take several weeks to simulate the motion of every atom in a polymer for just a few nanoseconds.
However, the force-extension curves from the computer simulations are in good agreement with the experimental data. And the advantage of simulations is that we can now explain the behaviour at the atomic or the submolecular level. The elastic contribution that is important at relatively small forces is due to the rotation of bonds between two carbon molecules that join the sugar rings (see figure 2c).
At some point during this rotation, the bond suddenly straightens and cannot be rotated any further. Any further elastic deformation can now only take place by flattening the sugar ring itself. Since this process involves several chemical bonds being deformed simultaneously, the effective stiffness of the polymer rises.
Although there are some quantitative differences between the experiment and the simulation – straightening the carbon-carbon bonds in the simulation requires a force that is twice as strong compared with the experiment, for instance – the overall agreement is very good. In fact, the level of agreement is remarkable when we consider that the computer simulation pulls the molecule nine orders of magnitude faster than the experiment.
So why should the “pulling speed” matter? We can think of the transition from one molecular shape to another as a two-level system with a so-called activation barrier separating the two states. When we pull the molecule with the AFM, we effectively reduce this barrier until it eventually disappears at a “threshold force”. But there is a finite probability that the molecule will cross this barrier due to thermal fluctuations, even when the force is less than the threshold value. The longer the molecule is held at a particular force, the more likely it is to change shape due a thermal fluctuation. This explains why the molecule makes this transition at a much lower force in the experiment compared with the simulation, which is significantly faster.
The elastic properties of other single polymer molecules were measured using an AFM in a similar way. Hermann Gaub and co-workers at the Ludwigs-Maximilians University in Munich have measured the elastic properties on poly-ethylen glycole, poly acrylic acid and deoxyribonucleic acid (DNA). The elastic properties of DNA are particularly important for cell replication and the synthesis of new proteins. In a cell, the DNA molecules are typically wound around little barrels called histons that are only about 10 nm in diameter. The DNA has to unwind before it can duplicate itself, and afterwards it has to rewind around the histons.
Specific interactions
There are many instances in biology where we need to know how much force a chemical bond can sustain. In drug development, for example, the effectiveness of a medication depends on the strength of the bond between a ligand in the drug and the receptor.
We can now measure the strength of an individual chemical bond in experiments using an AFM. In 1994 Ernst-Ludwig Florin, Vincent Moy and Hermann Gaub at Munich demonstrated this for the first time using a vitamin-B molecule called biotin, which reacts very strongly with a protein called streptavidin.

To measure the strength of this bond, the AFM tip was coated first with biotin and then with streptavidin. A bead-shaped substrate was also coated with biotin so that it would readily bond to the coated tip (figure 3a). Since a biotin molecule can bind to a streptavidin molecule at four different sites, bonds can be formed between the bead and the tip. The trick is to use very small amounts of biotin and streptavidin so that just one bond (or a few at the most) is formed when the AFM tip is brought into contact with the bead.
The adhesion force was measured as the tip was pulled away from the substrate until the bonds eventually broke. The Munich researchers found that the strength of an individual bond was around 200 pN. The rupture force changes when we use slightly different molecules, but simulations give us a detailed understanding of the actual processes that happen as the bonds break.
Several other groups have adopted this experimental scheme to measure the molecular rupture force for many other systems. Hans-Joachim Güntherodt’s group at the University of Basel in Switzerland measured the strength of the bond between various antibodies and their antigens, substances that can interact with specific chemical groups on the antibody. They also determined the force between proteoglycans – molecules that are important for the adhesion between cells.
Recently, Michel Grandbois in our group at Ludwig-Maximilians University in Munich demonstrated that the strength of a single covalent bond, the strongest type of chemical bond, could be measured (see Physics World June 1999). We have found that the rupture force of silicon-carbon bonds and sulphur-gold bonds is just a few nanonewtons. In biological terms, such large forces are not accessible using other sensitive single-molecule manipulation techniques, such as optical tweezers.
Structure and function of proteins
The beauty of an AFM is that we can investigate functioning processes within living biological systems, such as cells and single molecules. In 1994 Monika Fritz and co-workers at the Technical University in Munich observed the biological activity of human platelets (minute particles found in the blood) using an AFM. And at the single-molecule level, Monika Fritz, Paul Hansma of the University of California at Santa Barbara and I demonstrated that the activity of an enzyme that is present in milk and tears could be measured using an AFM. We showed for the first time that the activity of a single molecule could be determined directly using mechanical sensors. This may give important insights into how the shape and motion of molecules change as they carry out a biological function (see, for example, The physics of protein folding).

In the experiment the AFM tip was brought into contact with a dense layer of enzyme molecules that were adsorbed on a mica substrate. We kept the horizontal position of the tip stationary and monitored the height of the sample as a function of time by measuring the deflection of the cantilever (figure 4). We saw spike-like fluctuations only when the enzyme reacted with the substrate. No effect was seen when the enzyme was surrounded by a “buffer” solution so that it could not react with the substrate, nor when an inhibitor was added to destroy the activity of the enzyme.
Meanwhile, Magdalena Bezanilla and co-workers in Helen Hansma’s group at Santa Barbara followed how an enzyme called DNAse reacted with DNA that was adsorbed on a mica surface. They found that the enzyme digested the DNA molecules into smaller pieces until they disappeared entirely from the surface.
In 1997 Sandor Kasas and co-workers in the same group used an AFM to follow what happens in one of the first stages of protein synthesis. Living cells manufacture proteins from amino acids, according to the genetic information that is encoded in the DNA. Ribonucleic acid (RNA) acts like a messenger, carrying this information from the DNA to the protein factories within the cells. An enzyme called RNA polymerase reads the genetic information on the DNA strand and then synthesizes the complementary strand of RNA. Kasas and co-workers adsorbed DNA on a mica substrate and incubated it with RNA polymerase. Using an AFM, the researchers directly observed the polymerase move along the DNA strand one step at a time as it read the genetic information.
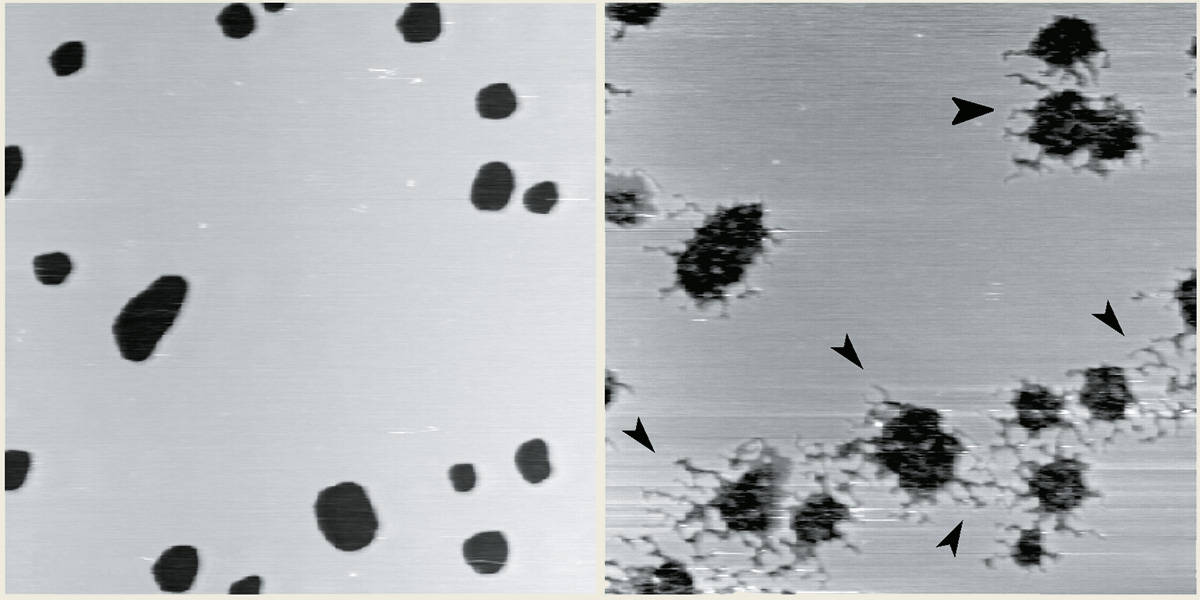
Meanwhile, Michel Grandbois and Hauke Clausen-Schaumann have studied the effects of phospholipase A2, the active ingredient in rattlesnake venom, with an AFM. When they added phospholipase A2 to a little patch of fatty-acid molecules that were adsorbed on a mica substrate to mimic a cell membrane, they saw the membrane being slowly digested. The activity of a single enzyme molecule was inferred from the creation of little canals in the patch as a single molecule digested its way through the fatty acid (figure 5).
Stability of proteins
Proteins are made from long, 1-D chains of molecules that fold up into certain 3-D structures. The detailed chemical and biological properties of the protein depend on how the molecule folds. Mechanical stability is very important for proteins that form muscle fibres, like myosin and kinesin, and for those that have to withstand forces (like cell-adhesion molecules that stabilize and form the contact between cells in tissues, for example).
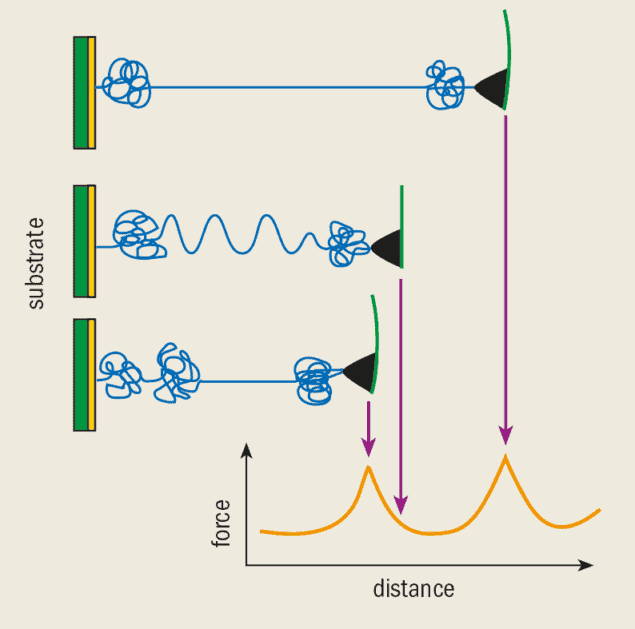
Muscle fibres contain two types of filament that slide against each other, causing the muscle to stretch or contract. A protein called titin acts as a buffer between the two filaments and protects them. But Harold Erickson at Duke University in the US has speculated that the mechanical properties of the titin molecule give the muscles their elasticity.
The structure of the protein molecule resembles a string of beads. The muscle’s elasticity may be due to either the random coils (the beads) or the “repeat region” made up of units called domains. The mechanics of the random coil has been determined by experiments using optical tweezers, whereas Mathias Rief and co-workers at Munich have investigated the properties of the domains using an AFM.
Short fragments of the titin molecule, either 4 or 8 molecules long, were first bound to a substrate. Rief then picked up the fragments using the AFM tip and pulled them until the individual domains unfolded (figure 6). The measured force increased as the polymer was stretched until one of the random coils unravelled. At this point the force dropped and the process continued, resulting in a force-extension curve with a saw-tooth pattern.
The titin force-extension curves are best described by the “worm-chain model” – another one of the standard models from polymer theory. In this model the elasticity of the polymer molecule is described by the energy needed to bend the molecule, rather than the energy required to rotate the bonds.
The experiment also determined the stability of a protein domain by measuring the force needed to unfold it. Simulations performed by Klaus Schulten and co-workers of the University of Illinois at Urbana Champaign showed that hydrogen “bridges” in the protein domain are important for its stability. By comparing experimental results with such simulations we gain a much clearer and more detailed understanding of the protein at the molecular level. Although the chemical and biological properties of dextran and titin are very different, the same physics underlies the elastic behaviour and can be understood by comparing the AFM results with a two-state model (figure 7).

Future outlook
The atomic force microscope has opened up a whole new class of experiments with single molecules in the physical and engineering sciences. But perhaps biologists have profited the most from the development the AFM, which allows them to study a wide range of living biological processes with unprecedented accuracy for the first time. In addition, AFM experiments give biologists the opportunity to study molecules in forms that are rarely seen in nature. In their natural habitat, molecules spontaneously change shape, and may even completely unfold and refold again. Conventional methods in biology examine an ensemble of many molecules, and therefore “average out” these shape fluctuations. However, by studying single molecules we can examine a given conformation in detail. Moreover, we can manipulate the molecules into states that are normally only present for a short period of time and study them in detail using an AFM.
Since atomic force microscopy is a relatively new technique, we can be sure of many more exciting developments that will undoubtedly shed light on the physics of life.