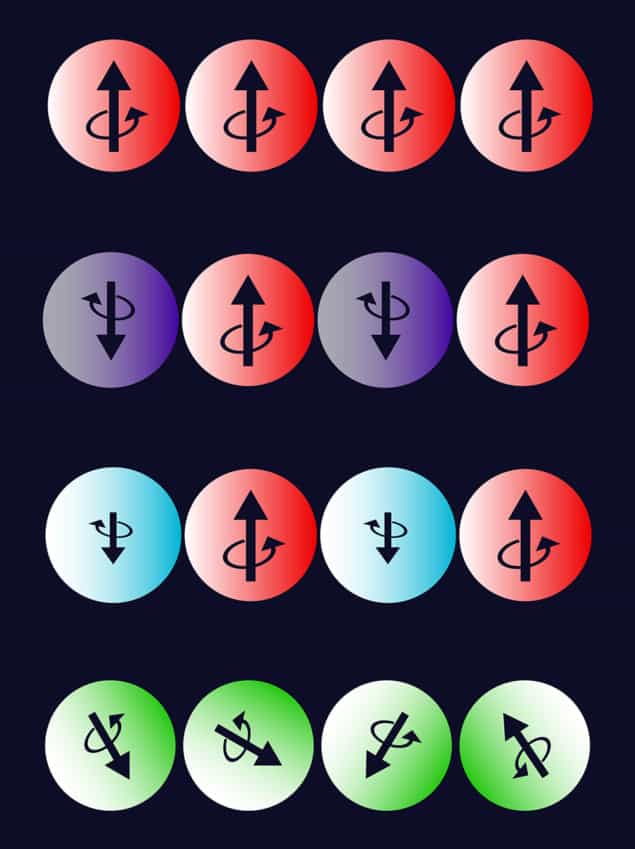
How do materials that are normally insulators manage to conduct electricity without resistance? Physicists have been debating this basic question about cuprates – ceramic compounds made up of layers of copper and oxygen atoms, interleaved with atoms of other elements – for more than three decades and have yet to come up with a satisfactory answer. Now, however, researchers in the US have put forward a model that at least provides clues as to what such an answer might look like, based on studies of so-called “overdoped” cuprates.
In 1986, Georg Bednorz and Alex Müller discovered that, by tweaking cuprates’ chemical composition, they could make these normally-insulating materials superconduct. What is more, the superconducting transition temperature of the doped cuprates far exceeded those of previously-recognized metallic superconductors – meaning that the established Bardeen–Cooper–Schrieffer (BCS) theory of low-temperature superconductors must be incomplete.
This finding led to much excitement, and subsequent studies succeeded in pushing the cuprates’ superconducting transition temperature up to around 150K. More recently, other materials – hydrides subject to very high pressure – have been found to superconduct at even higher temperatures. Yet the dream of room-temperature superconductivity remains elusive, and – frustratingly – physicists still haven’t agreed on a theory to explain the source of cuprates’ superconductivity.
Jumping electrons
Multiple studies have shown that cuprates superconduct when their electrons jump from one copper atom to another. Because electrons strongly repel each other, however, they usually exist in a static formation, with one electron sitting immobile on each atom. Undoped, therefore, cuprates remain in an insulating, antiferromagnetic state known as a Mott insulator, with neighbouring electrons having opposite magnetic spins.
When a cuprate is doped with atoms of other elements such as lanthanum, strontium or yttrium, some of its electrons are replaced with holes, freeing the remaining electrons to flow without resistance. Too much doping, though, and the effect reverses: beyond a specific concentration of dopant atoms, the cuprate’s transition temperature drops until it eventually becomes a metal. The latest research focuses on these “overdoped” cuprates, putting forward a model that aims to reconcile some apparently contradictory experimental results.
Contradictory behaviour
According to BCS theory, metals become superconducting when electrons partially overcome their mutual repulsion by creating what are known as Cooper pairs. These pairs form thanks to vibrations in the metallic crystal lattice: when one electron draws slower-moving ions towards it as it passes through the lattice, it generates a small concentration of positive charge that then pulls another electron in behind it.
Since electrons are fermions, they obey the Pauli exclusion principle, forming a Fermi gas in which the system’s energy levels fill from the bottom up with one electron per lattice site and spin. The boundary between the highest occupied energy level and the next is known as the Fermi surface, and the electrons that form Cooper pairs are those nearest this surface.
In a metallic superconductor, all electrons contribute to superconductivity, not just those bound up as Cooper pairs. In practice, this means that the density of superconducting electrons should be similar to the overall electron density. However, in 2016 Ivan Božović and colleagues at the Brookhaven National Laboratory in the US showed that this is not the case for overdoped cuprates. Instead, after devising a new way of preparing overdoped copper oxides (which are chemically unstable), they found that the density of superconducting electrons in these materials is actually far lower than would be expected from BCS theory.
Explaining the disparity
The new model seeks to explain this disparity. In doing so, it takes inspiration from work carried out by Japanese physicists Yasuhiro Hatsugai and Mahito Kohmoto. In the early 1990s, Hatsugai and Kohmoto proposed a way of overcoming the apparent contradiction between the statistics of a Fermi gas (which stipulate one electron per lattice site and spin) and a Mott insulator (which allow just one electron at each site). Their solution was to introduce what is, in effect, a new repulsive interaction that prohibits two electrons with opposite spins from occupying the same momentum state.
Philip Phillips and colleagues at the University of Illinois at Urbana-Champaign have now revised this model by adding a further interaction that draws electrons together to create Cooper pairs. As they report in Nature Physics, this model includes a Fermi surface, but also a much-reduced density of superfluid electrons compared with a normal Fermi gas – bringing it more in line with the observations made by Božović and colleagues at Brookhaven.
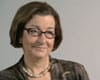
Life after the cuprates
Jan Zaanen of the University of Leiden in the Netherlands, who was not involved in the present work, points out that the Urbana-Champaign team’s modelled density falls off more gradually with increased doping than was observed in the Brookhaven experiment. Nevertheless, he believes that the new scheme provides a “proof of principle” for showing how a non-Fermi liquid could have a Fermi surface.
Since both Hatsugai and Kohmoto’s model and the Phillips’ group’s adaptation posit new interactions between electrons, while also describing those electrons as waves rather than particles, Zaanen acknowledges that it is hard to envisage them as real physical descriptions of what is taking place. However, he points out that a similar problem occurs in the well-established theory of Lev Landau that describes helium-3 and other systems in terms of strongly-interacting fermions.
As Zaanen explains, Landau’s scheme relies on emergent physics involving highly collective quasiparticles. A similar logic, he concludes, may be needed to understand overdoped cuprates. “Although nonsense on the microscopic scale,” he says, “the Hatsugai-Kohmoto interactions may spring into existence in the collectivization process to become eventually literal on the macroscopic scale”.