Technological devices are getting ever smaller, but as they approach the scale at which quantum physics matters, our understanding of how they interact with their environment evaporates. James Millen and André Xuereb explain how a better understanding of quantum thermodynamics could kick-start a new industrial revolution on the tiniest scale
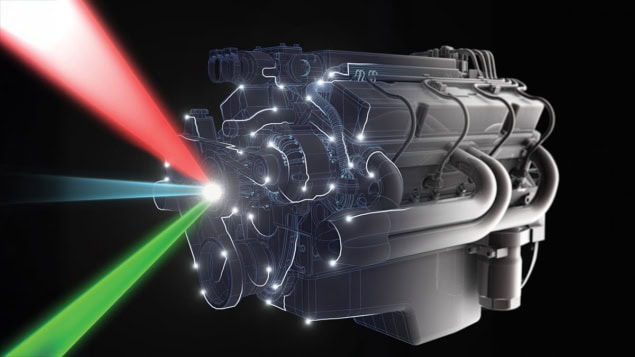
It is hard to overstate the impact of thermodynamics. This theory helps us understand immensely complicated systems – such as a gas containing billions upon billions of particles – using only a few basic quantities, notably temperature, pressure and volume. The study of thermodynamics led to the discovery that energy is conserved (the first law of thermodynamics) and that an absolute zero of temperature exists (the third law). It also introduced the concept of entropy, a measure of disorder, and showed that the entropy of the universe increases – meaning that things progressively tend to get messier and less ordered (the second law). Entropy defines the direction in which time flows: a glass of water will never “un-spill” or a firework “un-explode”.
On a more applied level, thermodynamics explains how to convert a form of waste energy (heat) into useful energy (work) – a principle that helped bring about the industrial revolution. Our modern world of internal combustion engines, refrigerators and electricity generation would not exist if we had not understood and made use of this incredibly fundamental, and yet immensely practical, theory.
But thermodynamics does not only apply to large objects. As you read this, air molecules are constantly hitting you at an average speed of about 1600 kilometres per hour. Sometimes one will hit you travelling at 2000 kilometres per hour, sometimes at a quarter of that. You don’t notice these rare deviations from the average because molecules are small compared with human beings. However, for tiny objects, such as the molecular “motors” inside living cells, these thermal fluctuations are extremely important. Any microscopic devices we build must also be able to handle these fluctuations without being destroyed or thrown around.
But what happens if we shrink machines further and enter the quantum world? In this realm there are not only thermal but also quantum fluctuations to deal with: even at absolute zero, where classical mechanics tells us that things should stand still, the uncertainty principle of quantum mechanics means that nothing is ever at rest. Worse still, quantum states are even more delicate than a molecular motor. A single gas molecule colliding with a quantum particle could destroy its quantum state, for example by causing an atom in a superposition of its ground and excited states to collapse into one state or the other.
To solve such problems, we need to understand how thermodynamics works on a quantum scale. Do the ordinary laws of thermodynamics still apply under these conditions? Do phenomena such as quantum entanglement affect the power or efficiency of quantum machines? Questions like these are the province of a new and rapidly evolving field called quantum thermodynamics, and we need better answers to them before “quantum engineers” can begin to build quantum machines. Such machines could have some very unusual properties: they might use entanglement as a fuel, for example, or be made from a single atom. And in the future, when even classical computers will operate at the level of individual atoms, quantum machines would be the natural way to power these super-small computers, control them and stop them overheating.
Creating quantum engines
In the quantum world as well as the classical one, engines are machines that convert one form of energy, such as electrical energy, into another, such as mechanical motion. It is hard to imagine our modern, classical world without such devices, so a logical first step towards building quantum machines is to build quantum engines.
Over the past few years, researchers have built several truly tiny engines. One such device, developed by Valentin Blickle and Clemens Bechinger at the Max Planck Institute for Intelligent Systems in Germany, involved micron-sized plastic spheres that were suspended in water and held in place by the force from a focused beam of light. By selectively heating the water surrounding the spheres with a laser, the researchers were able to drive a sphere’s motion like the piston of an engine.
Experiments like this have highlighted how important thermal fluctuations are in microscopic settings. They have also produced some seemingly counterintuitive results. For example, tiny objects immersed in a cold surrounding can occasionally take heat away from their environment. At first glance, such behaviour might seem to violate the second law of thermodynamics, since making something colder makes it more ordered; in terms of entropy, it is like a tiny glass of water un-spilling itself (figure 1). In fact, there is a loophole: in the microscopic world the laws of thermodynamics only hold on average. If we had lots of tiny glasses of water, most of the water would tend to stay spilt, with only a few glasses un-spilling themselves. Thus, the second law of thermodynamics holds when applied to all the glasses as a group.
1 Un-spilling a tiny glass of water
When a glass of water is spilled (left) it is essentially impossible that all of the water molecules will spontaneously run back into the glass. However, in a microscopic glass of water that contains only one water molecule (right), random thermal fluctuations mean that it is possible that a single molecule will jump back into the glass.
Tiny engines help us to understand these effects, but as small as they are, they are not yet operating in the quantum world. So is it possible to build a truly quantum engine? One key point is that to operate, an engine needs to be connected to a hot environment (such as exploding fuel) and a cold one (such as the outside world). Then, as heat flows from the hot environment to the cold, work can be extracted. This sounds simple enough, but in the quantum world, being in contact with the environment is tricky, since quantum states are extremely sensitive to disturbances from their surroundings. Since a collision with a single air molecule can be enough to destroy a quantum state, connecting a quantum engine to its environment without destroying its “quantumness” seems a daunting task.
Recent research by several groups in optomechanics (the study of how light interacts with moving matter) has suggested various exciting ways of getting around this problem. One promising approach is to shine a beam of light on a single ion that has been isolated from its environment by being placed in a vacuum and suspended using an electric field. Some frequencies of light will cause the ion to move faster, thereby heating it, while others will slow it down, cooling it. These different frequencies of light can therefore be thought of as environments at different temperatures. The result would be an ion that moves back and forth like a piston in a car engine, similar to the microscopic spheres in Blickle and Bechinger’s work (figure 2).
2 A quantum ion engine
(Courtesy: James Millen and André Xuereb)
One proposal for building a quantum engine (put forward by researchers at the universities of Mainz and Erlangen, Germany) involves trapping single ions and then driving their motion like a piston using different frequencies of light. The ion would be trapped by electric fields provided by the angled rods shown in this image, and the light would act like a quantum environment, with the frequency determining its temperature.
Other ideas involve trapping light in a cavity between two parallel mirrors. When light reflects off a mirror, it exerts radiation pressure that pushes the mirror away from the light source, and this pressure could in principle be used to move one of the parallel mirrors like a piston. Alternatively, the light in the cavity could drive the motion of a quantum object such as an atom.
Efficiency enhanced
A key quantity we often hear about in practical thermodynamics is the efficiency of an engine – that is, the amount of useful energy the engine puts out for a given amount of energy put in. The point at which an engine is most efficient, known as the Carnot limit, is frustratingly also the point at which it produces the least power. A maximally efficient car, in other words, wouldn’t actually be able to move. However, quantum physics allows us to play tricks, and recent research by groups at the universities of Erlangen and Mainz has shown that a quantum engine should be able to beat the standard Carnot limit and yet still produce power. One way in which this is achieved is via “quantum squeezing” – a technique in which we come to know something about an object (such as its location) much more precisely, at the expense of knowledge about something else (such as how fast it is moving). Squeezing certain parameters of the environment will make it appear, in effect, hotter or colder than naively expected, and since the efficiency of an engine depends on the ratio of the environmental temperatures, an engine in contact with a squeezed environment may outperform the Carnot limit (figure 3).
3 Feeling the squeeze
Quantum engines can be more efficient than classical engines. (a) A diagram showing the evolution of an Otto engine, such as the spark-ignition piston engine commonly found in cars. Work, represented by the area inside the cycle, is extracted by heating gas (for example by burning fuel) to drive a piston. The position x and momentum p of the piston follow the rules of thermodynamics, and will most likely be found within the circles shown on the inset graphs. (b) A quantum Otto cycle. By “squeezing” the quantum piston – where the term “squeezing” means knowing where it is more precisely, at the expense of knowledge about its momentum – we can extract more work than the classical case, as shown by the larger area within the cycle.
A quantum car might sound like good value for money, but there is a price to pay. We need to do more research to understand how to preserve the delicate nature of quantum states once they are deployed to power machines. Intriguingly, one possible application for quantum engines would be to produce or maintain an environment suitable for nearby quantum devices. For example, since heat easily destroys quantumness, a “quantum refrigerator” – a device that removes excess heat generated by a quantum machine – would be extremely useful. Amazingly, research by Noah Linden, Sandu Popescu and Paul Skrzypczyk suggests that a fully working refrigerator could be made out of a single atom. They pictured an atom with three internal energy states, immersed in an environment where two of the three states interact with a hot bath, and two with a cold bath. The researchers showed that this single atom may take heat away from the cold bath and dump it into the hot bath – just like a normal household refrigerator does.
Finding quantum analogues
Quantum physics is a theory rife with probabilities, offering a huge landscape of possibilities. The laws of thermodynamics, in contrast, place strong limits on what can actually happen in the quantum world
Quantum physics is a theory rife with probabilities, offering a huge landscape of possibilities. The laws of thermodynamics, in contrast, place strong limits on what can actually happen in the quantum world. If quantum physics were a game of football, quantum thermodynamics would dictate who is allowed to play (zeroth law), how you’re allowed to kick the ball (first law), whom you can pass to (second law) and how long the game will last (third law).
Marrying these two very different theories is challenging, but as it turns out, many of the powerful rules from classical thermodynamics have analogues in the quantum world, albeit in different forms. Jonathan Oppenheim, a physicist at University College London and an expert in fundamental aspects of thermodynamics at the microscopic level, explains that not only does the second law of thermodynamics hold for quantum systems, there is in fact an entire “family” of second laws. These additional second laws tell us that systems become more disordered, Oppenheim says, but each one also constrains the way in which they become more disordered.
For example, one way of phrasing the ordinary second law of thermodynamics is to say that the Helmholtz free energy, H, of a system (defined as the difference between the system’s internal energy U and the product of its temperature T and entropy S; H = U – TS) must decrease. Together with colleagues from Poland and Singapore, Oppenheim showed that in microscopic systems, a whole family of free energies exists, and all of these free energies must decrease. At the scale of ordinary objects, these other free energies become identical and, as Oppenheim concludes, “all the quantum second laws are equal to the one we know and love”.
Another important link between quantum physics and thermodynamics is that they are both theories of information. In quantum physics, the process of measurement, which basically means collecting information, plays a central role. For example, suppose you have an electron in a uniquely quantum superposition of being in two places at the same time. As soon as you try to measure, or gain information about, its position, the electron’s “quantumness” vanishes, and you will always find it in one place. The very act of gaining knowledge about a quantum state may change it irreversibly.
In thermodynamics, information plays a similarly important role. In 1929 Leo Szilard described how knowledge itself could be exploited to generate useful energy. In his “Szilard engine” thought experiment, he reasoned that if you measure where the hot gas is in an engine, you can then make the best decision about where to put a piston to drive the machine, thus extracting work from information. Some three decades later, another physicist, Rolf Landauer, observed that it also takes energy to forget things, and thus there is a cost to holding information. In fact, if all the computer servers in the world forgot all the information stored on them, one could, in theory, extract enough energy to run a light bulb for a few hours – not a lot of energy, but amazing considering that the only fuel is knowledge.
A quantum battery
Landauer’s principle and Szilard’s engine are both creatures of the classical world. So can we also extract useful energy from quantum information? Recent research by Lídia del Rio of the University of Bristol, UK, suggests that the answer is yes. In fact, by using quantum information (specifically quantum entanglement) as our “fuel”, it is possible to extract more energy, and do so more efficiently, than we can in a classical system.
Quantum information is stored more densely than classical information: if we flipped a quantum coin, the outcome could be heads, tails, or heads and tails
The origins of this conclusion lie in the fact that quantum information is stored more densely than classical information: if we flipped a quantum coin, the outcome could be heads, tails, or heads and tails. This is closely related to the phenomenon of quantum entanglement, and could have practical benefits, says John Goold, an expert in understanding complicated quantum systems who is based at the International Centre for Theoretical Physics in Trieste, Italy. Together with co-workers from the UK, Singapore and Australia, Goold considered the route through possible energy states that a cell in a battery has to take when it goes from being fully discharged to fully charged. When several such cells are combined into a battery, the route becomes much shorter when the intermediate state of the battery is entangled. In practical terms, this implies that a quantum battery would take significantly less time to charge than an ordinary one. According to Goold, further research into quantum batteries may help us develop novel materials that could function as the energy storage media of the future.
Building understanding
Quantum technologies are at the cutting edge of science and engineering, and the concept of a quantum engine is newer still. Researchers are only just beginning to understand the applications and limitations of these devices. The nature of the laws of thermodynamics and concepts as basic as heat and work at the microscopic scale are still under debate. Work on how to protect very delicate quantum states in any realistic quantum device is ongoing. But with unbreakable cryptography and teleportation already a reality, and quantum computers ever nearer, mastering quantum thermodynamics, and the quantum machines it describes, will be an invaluable step in helping to bring these new technologies to life.