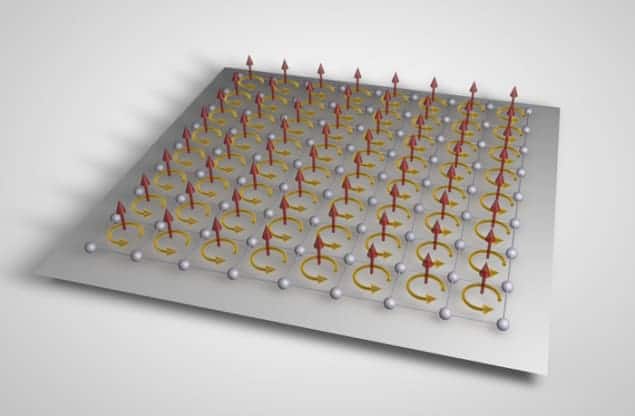
The elusive Hofstadter’s butterfly could soon be spotted in lattices of ultracold atoms, now that two groups of researchers have independently created the conditions required for a spectacular fractal pattern to emerge from the energy spectra of ultracold rubidium atoms held in optical lattices. Although neither team has directly observed the fractal pattern, they have created physical systems with the right conditions for Hofstadter’s butterfly to emerge. The research could also lead to the development of new ways to simulate quantum systems with exotic electric properties.
In 1976 the American physicist Douglas Hofstadter – famous for the 1979 book Gödel, Escher, Bach – first outlined the concept of the butterfly that bears his name. He predicted that stunning self-similar patterns now known as “fractals” would arise in the energy spectrum of electrons in crystalline solids exposed to extremely large magnetic fields. Due to the periodic nature of the electric fields in a crystal, the electrons are restricted to series of energy bands. When a magnetic field is applied to electrons inside a crystal, their motion is modified by the Lorentz force and they move around in circles. Hofstadter calculated that as the magnetic field becomes stronger, the energy bands split again and again, producing a butterfly-like energy spectrum.
Theoretical curiosity
The concept made an unexpected and exciting link between quantum mechanics and mathematics. However, impractically large magnetic fields are required to see the effect in conventional solids, so for years the butterfly was a theoretical curiosity.
Then in 1998 Ulrich Kuhl and Hans-Jürgen Stöckmann of the University of Marburg in Germany designed a microwave experiment that simulated the conditions required for Hofstadter’s butterfly to emerge. The approach that they took is known as quantum simulation, whereby the physics experienced by electrons in a solid is mimicked using another physical system. Then in May 2013, the fractal pattern was measured experimentally for the first time in a real solid – graphene on a boron-nitride surface.
The two latest studies are also quantum simulations but mimic electrons in a solid using ultracold neutral rubidium atoms trapped in a lattice made of criss-crossing laser light. Both teams begin their experiments by cooling a cloud of rubidium atoms to a chilly 100 nK. This creates a Bose–Einstein condensate (BEC) in which all the particles behave as a single particle. “Then we load these particles into an optical lattice, which is simply a periodic structure of bright and dark areas created by the interference of counter-propagating laser beams,” says Monika Aidelsburger, a member of the team led by Immanuel Bloch at the Ludwig-Maximilians-Universität Munich and Max-Planck Institute of Quantum Optics in Germany. “As a result, the system of neutral atoms in the optical lattice simulates a real material: the neutral atoms behave as electrons and the optical lattice mimics the periodic crystal structure.”
Giving atoms a kick
To simulate the effect of a magnetic field, the team used a pair of criss-crossed laser beams that “kicked” the atoms as they move across the lattice, making them tunnel from one lattice site to another. “If the atoms move from left to right, they get a kick in one direction, but if they move from right to left they get a kick in the opposite direction,” Aidelsburger explains. “These kicks resemble the Lorentz force felt by electrons when moving with positive or negative velocity, and therefore allow for the simulation of a real magnetic field.”
“Our atoms behave as electrons in a real material under the effect of a magnetic field, with the difference that we do not apply a real magnetic field,” says Julio Barreiro, who is also a member of Bloch’s team.
The other team, led by the Nobel laureate Wolfgang Ketterle at the Massachusetts Institute of Technology in the US, has obtained exactly the same results. “The real fundamental quantity is the quantum mechanical phase the wavefunction accumulates as it moves around in the system, so by imprinting this phase onto our atoms we can simulate the effect of magnetic fields unattainable in conventional condensed matter systems,” says Ketterle’s colleague Colin Kennedy.
Studies of real materials
The results may pave the way towards solving quantum mechanical problems with many interacting particles by using quantum simulation, says Kennedy. “Our work provides a crucial step toward simulating the physics of charged electrons in a magnetic field, which constitute real materials.”
An important feature of the technique is that the synthetic magnetic field can be easily adjusted. As a result the method “will offer the possibility to investigate the physics hidden in the fractal butterfly in a unique and very controllable way,” explains Nathan Goldman of Laboratoire Kastler-Brossel in France, who was not involved in the studies.
Anatoli Polkovnikov and Claudio Chamon of Boston University say that both experiments also offer a new way of simulating strong magnetic fields. “One can imagine that these fields can be made time-dependent generating artificial electro-magnetism, which was never studied before,” they say. “It is hard to anticipate all applications from exploring new, not very well understood regimes – very likely there is potential for unexpected discoveries.”
Both studies appear together in the journal Physical Review Letters: Phys. Rev. Lett. 111 185301 and Phys. Rev. Lett. 111 185301.