Recent experiments performed on ultralow-temperature plasmas created from laser-cooled atoms are unleashing a flood of new ideas in atomic physics.
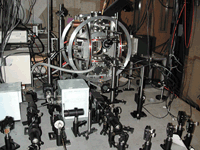
During the past decade, the availability of simple, effective methods for laser cooling and trapping has enabled enormous advances in the experimental study of atoms in their ground state. Until quite recently, however, these techniques have had much less impact on investigations of highly excited atoms – and almost none on plasmas. Several laboratories have now begun experiments in which atoms are initially prepared in the ground state at sub-millikelvin temperatures and then excited by a laser to very high electronic states, or even ionized. The result is a gas in which the atoms are in a highly excited state but move very slowly. This unusual scenario is, in reality, quite attainable – although the system is intrinsically unstable.
Now Thomas Gallagher of the University of Virginia in the US, Pierre Pillet of the Laboratoire Aime Cotton in Orsay, France, and co-workers have reported a remarkable manifestation of this instability. They have found that a sufficiently dense sample of highly excited cold atoms ionizes spontaneously with very high efficiency. In other words, the sample of gas converts itself to an extremely cold plasma in a matter of microseconds (M P Robinson et al. 2000 Phys. Rev. Lett. 85 4466).
Remarkable properties
The preferred internal energy state of a cold atom is the state with the lowest energy (i.e. the ground state). Laser radiation can promote the atom to higher-energy states, or even remove the electron altogether by the process of photoionization. High-energy states, in which the electron is barely bound, are known as Rydberg states, and these have many remarkable properties. For example, the electron is very far from the nucleus.
If we label each state by its principal quantum number n, where n is large for Rydberg states, then the characteristic radius of the electron’s orbit around the nucleus scales as n2, increasing from ~0.05 nm for the ground state to over 100 nm for a state with n = 50. The size of such an atom is comparable to the smallest feature on a modern integrated-circuit chip.
In contrast, the energy needed to remove the electron from the atom scales as 1/n2, decreasing from several electron-volts for the ground state to about 5 millielectron-volts for n = 50. Due to their small binding energy, Rydberg states tend to be very fragile and sensitive to external perturbations such as collisions or electric fields.
If the electron has sufficient energy, it can leave the atom, yielding a free electron and a positively charged atomic ion. A collection of such negative and positive charges is known as a plasma – a state of matter that appears in such diverse places as astrophysics, thermonuclear fusion, fluorescent lighting and semiconductor processing (see Technological plasmas in this issue by Bill Graham).
In thermal equilibrium, the temperature of a plasma must be high enough for the thermal energy to exceed the binding energy of the atoms. This requires temperatures of the order of 10 000 K, otherwise the positive and negative charges are perfectly happy to recombine into bound atoms. However, recent work with laser-cooled atoms has shown that very-low-temperature plasmas can be created – and can also survive long enough to be studied experimentally.
Several recent experiments have used a laser-cooled gas as the starting point for creating an ultracold plasma. Steve Rolston’s group at the NIST laboratory in Gaithersburg, US, for example, has given the electrons in ultracold xenon atoms just enough energy to ionize them with a laser (T Killian et al. 1999 Phys. Rev. Lett. 83 4776). Since the electrons are so light, they carry away the majority of the energy, leaving the ions almost as cold as the initial atoms. The liberated electrons begin to disperse, but only a small fraction leave the gas: the residual net positive charge is enough to trap the remaining electrons, allowing the formation of a relatively stable ultracold plasma. Subsequent experiments by Rolston’s group have investigated the slow expansion of this plasma, as well as the oscillations that can be excited in it by radio waves.
Plasma avalanche
In one of the most surprising recent developments, the Virginia-Orsay team has observed that such an ultracold plasma can form spontaneously from a gas of ultracold Rydberg atoms. In the experiments, ultracold rubidium or caesium atoms with temperatures in the range 140-300 microkelvin are excited with a pulsed laser into highly excited Rydberg states with n = 35-40 (see figure 1). A gas of Rydberg atoms can be distinguished from a plasma by the electric field that must be applied in order to extract charges from the sample. For weakly bound Rydberg atoms, the field must be large enough to rip the electron from its atom. In a plasma, on the other hand, the electrons are already free.
By varying the initial number of Rydberg atoms, Gallagher, Pillet and co-workers observed a threshold above which the sample rapidly converts from Rydberg atoms to a plasma on a timescale of a few microseconds (see figure 2). They explain this avalanche plasma formation as a three-step process. First, a small number of the cold Rydberg atoms are ionized – either by collisions with the small number of room-temperature Rydberg atoms present, or by absorbing background radiation. A few of the electrons that have been produced escape, but the remainder are trapped by the net positive charge to produce a very dilute ultracold plasma that coexists with the remaining Rydberg atoms. Finally, these trapped electrons move throughout the Rydberg sample, colliding with and ionizing the very fragile atoms. The more free electrons that are available, then the more rapid the production of new electrons – hence the avalanche nature of the process.
Puzzling phenomena
An interesting question arises. Where does the energy come from? The initial Rydberg atoms have negative energy because they are bound, while the nearly neutral plasma state has positive energy. Energy is clearly required to produce the initial electrons that “seed” the avalanche process – this comes from the initial ionization process, either collisions or absorption of radiation. However, every time an electron ionizes a Rydberg atom, it must give up a significant amount of energy. So how can the process be sustained?
A likely possibility is that electrons sometimes undergo “super-elastic” collisions, in which an excited atom gives up part of its electronic excitation and the electron departs with increased kinetic energy. Clearly further work is needed in order to address this important issue.
In our lab at the University of Connecticut, we have recently observed not only the formation of the avalanche plasma, but also the plasma state reverting back into bound Rydberg states. In other words, we start with a sample of Rydberg atoms that ionize in a few microseconds to form an ultracold plasma. But, if we wait long enough – about 20 microseconds – we actually begin to see Rydberg atoms again. These atoms are presumably the result of three-body recombination in which two electrons and an ion collide to leave one of the electrons bound in a Rydberg state, while the other electron takes away the binding energy. It seems that after a brief period of separation, the electrons and ions are happy to be reunited back into Rydberg atoms. Rolston’s group at NIST also sees this type of recombination, although they start with a plasma that has been produced directly by the laser photoionization of ground-state atoms. The physical basis of this recombination is not yet fully understood.
Ultracold plasmas are generating interest because of the possibility that they are “strongly coupled”, meaning that the potential energy of neighbouring charges exceeds the typical kinetic energy. In other cold plasmas composed solely of trapped ions this has been shown to lead to a type of crystallization. Moreover, recombination of a low-temperature positron-antiproton plasma has been discussed as a way of efficiently forming antihydrogen atoms.
The theoretical treatment of ultracold plasmas is also of considerable interest. A rethinking of traditional collisional models is required because, at such low temperatures, the ions hardly move at all on the timescale of the ionization and recombination processes. We must think more in terms of interactions rather than of collisions.
The ultracold Rydberg atoms themselves are interesting because of their strong interactions and the possibility of observing collective effects. Schemes for quantum logic gates using ultracold Rydberg atoms have also been proposed. The recent marriage of highly excited atoms and ultralow temperatures has already unveiled some surprises – others undoubtedly await to be uncovered.