Michael Allen looks at a novel fusion experiment that promises a “steady state” plasma
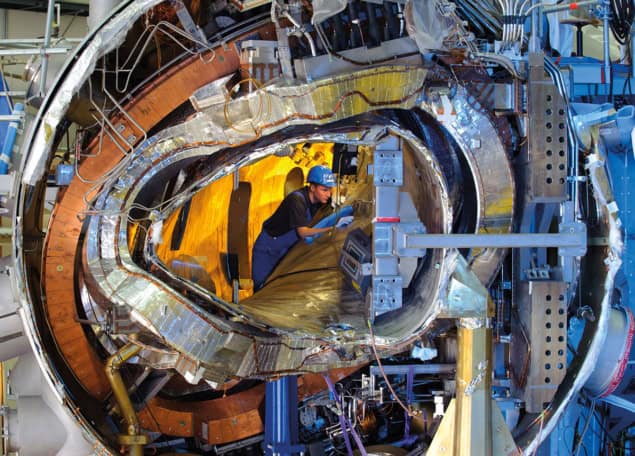
In February 2016 German chancellor Angela Merkel made a special trip to Greifswald – a small city in the north-east of the country next to the Baltic Sea. Merkel, who has a PhD in physics, was personally on hand to usher in a new era of plasma physics as she switched on the €1bn ($1.1bn) Wendelstein 7-X fusion device. Built on the outskirts of the city at the Max Planck Institute for Plasma Physics (IPP), Merkel’s visit marked the start of the reactor’s scientific run as the device burned its first hydrogen plasma at a temperature of 80 million degrees for about a quarter of a second.
Following the successful start of operations, Merkel declared that Wendelstein 7-X is “a unique experiment” that could “take us one step closer to the energy source of the future”. “[It] is the world’s most important fusion device of the stellarator type,” Merkel noted, adding that experiments could provide “important insights into whether stellarators can one day be used for the commercial production of energy”.
While experimental fusion devices have been around for decades, most of them – such as the ITER reactor currently under construction in France – are tokamaks. Stellarators and tokamaks both use magnetic fields to confine a plasma, but how they do this is different. To hold the plasma in place, the particles in the plasma need to be driven through a helical pattern. A tokamak uses toroidal magnets to generate a magnetic field that travels around the tokamak. This is combined with a vertical looping magnetic field generated by an electrical current induced in the plasma by a transformer. But as transformers only work in pulses, it makes it very difficult to generate a steady-state plasma – the plasma can collapse in between the pulses.
Stellarators, however, only use magnetic fields generated outside the plasma. This means that the magnetic fields can be continuous, in theory making it easier to generate a steady-state plasma. Yet stellarators are fiendishly difficult to build as the use of external magnetic fields means that, compared with tokamaks, a different plasma shape is needed. The optimal plasma for the latest generation of stellarators resembles (when viewed from above) a pentagon, varies in cross-section and moves through a more-pronounced twisting pattern than in a tokamak. Generating a magnetic field that can create these patterns requires magnets with a complex 3D geometry that need to be as close as possible to the plasma. Because of this, the inside of the reactor is far from a smooth torus like a tokamak – it is an incredibly complex 3D shape. Indeed, building Wendelstein 7-X has proved challenging, with the project costs doubling from the original €500m estimate.
The next generation
The first stellarator was developed at Princeton University in the US by physicist Lyman Spitzer in the 1950s. It created the helical pattern by confining the plasma in a figure-of-eight-shaped tube. Later designs used superconducting coils – in combination with toroidal coils – that spiralled around a doughnut-like tube to generate the required magnetic field. Before Wendelstein 7-X came online earlier this year, the world’s largest and most successful stellarator was the Large Helical Device in Toki, Japan, which had a performance approaching that of a similarly sized tokamak. It began operating in 1998 and uses two superconducting helical coils to twist the plasma.
The first “advanced” stellarator was Wendelstein 7-AS, which operated at the IPP’s institute in Garching, Germany, from 1988 to 2002. In the US, the helically symmetric experiment (HSX) was built at the University of Wisconsin-Madison, and started operating in 1999, while in 2004 researchers at the Princeton Plasma Physics Laboratory started building their own larger device – the National Compact Stellarator Experiment (NCSX). However, because of spiralling budgets it was cancelled four years later with the project struggling to assemble the various 3D sections and magnets with the required precision.
Wendelstein 7-X is part of a new generation, and rather than relying on helical and toroidal magnets, it uses complex 3D superconducting magnets to confine and twist the plasma.
Indeed, theoretical physicists calculated the best shape for the plasma and so derived the basic shape of the coil that can produce the desired magnetic field. The computing power required to calculate the optimal shape of the magnetic field and magnets needed to generate it was not available until the 1980s.
Complex design
During the construction of Wendelstein 7-X, it took about 12 years to perfect the design of the magnets and build a suitable prototype. Physicists and engineers went back and forth until they reached a compromise between coil shapes that could be manufactured and the optimal plasma shape. The main sticking point was the minimum radius through which the superconducting coils could be bent. Engineers also had to work out how to construct magnets with these complex 3D coils at the centre.
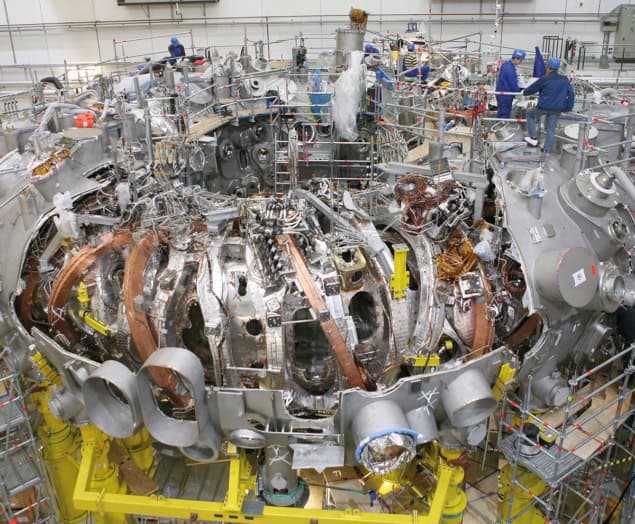
The construction of the magnets is similar to those on tokamak reactors. A niobium–tin superconductor is embedded inside a cable of copper wires – during operation this is cooled with liquid helium to a temperature of 4 K. Most superconductors enclose this cable in a stainless-steel jacket, but Wendelstein 7-X uses aluminium jackets to provide the flexibility needed for the complex 3D shapes. The conductor is then wound through 108 turns to create the magnetic coil and enclosed in a steel case filled with hardened sand and resin. The final 3.5 m-high magnets weigh six tonnes.
While a similar-sized tokamak device would need around 18 magnets, Wendelstein 7-X has 50 primary “bean-shaped” magnets. A stellarator is normally much lower and wider than a tokamak, which, assuming the same plasma volume, gives it a much larger circumference. According to Thomas Rummel, who managed the magnet production, this requires more magnets to avoid gaps between coils that could be detrimental to the magnetic-field stability.
The final design for Wendelstein 7-X uses five sets of 10 magnets. Each set has a different geometry and is on a different electrical circuit so the magnetic fields can be adjusted separately. This is necessary because the plasma does not travel around a perfect circle. Wendelstein 7-X also has 20 flat secondary superconducting magnets on two electrical circuits. These are not absolutely necessary for operating the stellarator, but provide “flexibility to allow a broader range of plasma parameters”, notes Rummel. This will allow researchers to investigate the physics of the plasma in more detail.
One big step
While stellarator research is set to be hugely improved by Wendelstein 7-X, which hopes to maintain a plasma for around 30 min, it is still behind that of conventional tokamaks such as ITER, and it may never reach parity. “Unfortunately, stellarators are one big step behind tokamaks and are not yet in a position to think about power generation,” says Rummel. “Our research over the next year will concentrate on finding the best plasma shape, holding the plasma stable and trying to find clever ways to bring the energy out.”