New experiments are pushing our understanding of light and optics to the limit and are also opening up new areas of physics and novel applications.
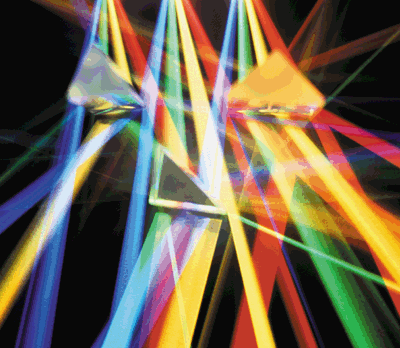
Optics has always been the most pure and the most applied area of physics. This is as true now as at any time in history. From Newton and his prism to the semiconductor lasers and optical fibres that form the modern world’s communications infrastructure, light has both fascinated physicists and facilitated whole new industries.
In common with all applications of cutting-edge science, fibre communications, medical imaging and other examples of optical technology rely on light behaving in a highly predictable fashion. The light – be it considered a wave or a particle or a ray – must obey the laws of optics. But rules exist to be broken, or at least bent, and as the articles in this special issue illustrate, optics is no exception.
Enter the laser
The invention of the laser in 1960 revolutionized optics and that impact is still being felt today. Modern laser research progresses on many fronts: some physicists want higher intensities and pulse energies, while others are busy trying to increase the efficiency of semiconductor lasers. On page 41 Ferenc Krausz describes research at another frontier – the quest for ever-shorter pulses (see print edition). However, at optical wavelengths, a 5 femtosecond pulse, say, will contain only two or three complete cycles of the laser field. But many processes – such as the movement of electrons inside atoms – occur on timescales that are even faster than this. Researchers must therefore resort to shorter wavelengths and a variety of nonlinear techniques to access the attosecond regime.
Shorter wavelengths are also essential for better resolutions in imaging applications. Indeed, for many years it was thought that the resolution of any optical instrument was limited to about one-third of the wavelength of the radiation being used. Initially the scanning electron microscope, which exploited the much shorter de Broglie wavelengths of particles, was used to reach the parts that optical microscopes could not access. Atomic-force and scanning-tunnelling microscopes followed, but light still has a role to play in the quest for molecular resolution, as Vahid Sandoghdar explains on page 29 (see print version). For instance, by using single molecules as light sources in so-called scanning near-field optical microscopes, it has been possible to achieve a resolution of 180 nm, and for certain applications resolutions of 10 nm or so have been obtained.
Light travels at 299 792 458 metres per second in a vacuum. Not surprisingly it slows down when it encounters anything, be it a piece of glass or a gas of sodium atoms. But if the sodium atoms have been placed in a particular quantum state by a “coupling” laser, it is possible to significantly slow down pulses from a second “probe” laser operating at a carefully chosen wavelength. And as Lene Vestergaard Hau explains in her article, under certain conditions it is possible to reduce the speed of the probe pulse to zero! This trick depends on the variation of the refractive index of the gas with wavelength. Remarkably, a similar trick can be used to increase the speed of the probe to hundreds of times the speed of light (Physics World September 2000 p21).
How do these tricks work? Basic optics teaches us that the speed of light changes from c to c/n when it enters a material with a refractive index of n, while the wavelength changes from L to L/n. We are also taught that the refractive index is also a function of wavelength or frequency. However, when a pulse of light – which must, by definition, contain a range of wavelengths – enters a material, it is slowed down by factor that depends on the variation of the refractive index with frequency. Controlling this variation is the key to changing the speed of the probe pulse.
Basic optics also teaches us that the refractive index causes a ray of light to be bent towards the normal. However, as John Pendry explains on page 47, this is not always the case, and in recent years it has become possible to build structures with negative electric and magnetic properties (see print version). Strangest of these are materials that have a negative refractive index. The new materials emerging from this research could lead to applications in communications, electronics, medical imaging and other branches of optics, and include the possibility of the “perfect lens”.
And there’s more
These are just four areas in which physicists are pushing the laws of optics to the limit. There are many more. Last month, for instance, physicists reported that they had built an optical clock that should be substantially more accurate than the world’s best atomic clocks. Moreover, the new clock relies on the application of a piece of totally unrelated physics research – the discovery of photonic crystal fibres – that is only two years old. Other recent advances include the first all-optical technique for making a Bose condensate and proposals to use “entangled” photons to improve the performance of laser-ranging and lithographic equipment.
Clichés about bright futures and the light fantastic abound in magazine articles about lasers and optics. But for once the clichés are actually true: the future for lasers and optics is very bright indeed.