The latest images of the cosmic microwave background reveal the seeds of galaxy formation.
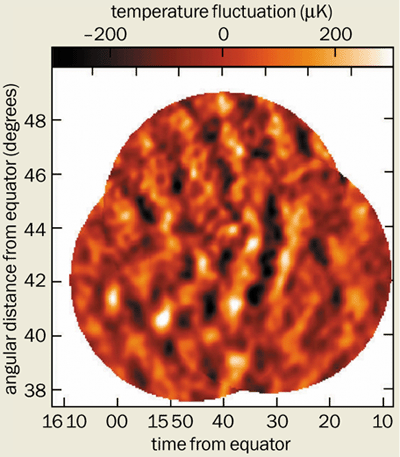
Recent observations of the microwave radiation emitted shortly after the Big Bang reveal that the face of the universe has a familiar pattern. The observations were made using two arrays of radio telescopes – the Cosmic Background Interferometer (CBI) in Chile and the Very Small Array (VSA) in Tenerife. The experiments have produced the sharpest measurements ever of the temperature variations in the cosmic microwave background. These variations trace the fluctuations in the distribution of primordial matter that seeded the formation of large-scale structure in the universe. The latest observations show that the angular distribution of the fluctuations agrees perfectly with previous data. More importantly, the results extend previous measurements to allow further detailed tests in cosmology (T Pearson et al. 2002 arXiv.org/abs/astro-ph/0205388 and A Taylor et al. 2002 arXiv.org/abs/astro-ph/0205381).
Conditions in the early universe
According to the standard model of cosmology the universe started with a Big Bang some 15 billion years ago followed by a brief period of very rapid expansion known as inflation. During inflation the primordial quantum fluctuations that existed at the time of the Big Bang were amplified into macroscopic fluctuations in the density of the universe – fluctuations that eventually formed the large-scale structure of galaxies that we observe today.
After inflation the universe continued to expand, albeit more slowly, and also to cool. During this time the light created at the Big Bang was constantly being scattered by the free electrons in a plasma of primordial hydrogen and helium ions. About 300 000 years after the Big Bang the universe cooled sufficiently for electrons and protons to form atoms, and the scattering stopped. Today these photons form a black-body spectrum with a temperature of about 3 K.
However, this cosmic microwave background is not completely uniform and has slight variations in temperature. These variations – known as the anisotropies of the cosmic background – reflect the slight differences in the density of the universe that existed at the time of “last scattering”. At this time the size or horizon of the universe was about 100 megaparsecs (about 300 million light-years). The surface of last scattering is now about 5000 megaparsecs away from us, which means that the horizon subtends an angle of approximately 1 degree.
Measurements of the anisotropy of the cosmic background are generally plotted as the amplitude of the temperature fluctuation against the angular scale, θ, or spherical harmonic, l, where l = 180/θ. The aim is to measure the temperature as accurately as possible and with the best possible angular resolution – that is with the smallest θ or the largest l.
In the early 1990s the COBE satellite measured the temperature with an accuracy of 1 part in 100 000 and an angular resolution of 7 degrees – results that strongly supported the theory of inflationary cosmology. Less than 10 years later, cosmologists were basking in the glory of higher-resolution measurements from three other microwave background experiments – the balloon-based BOOMERANG and MAXIMA telescopes, and the DASI interferometer located at the South Pole. These observations enabled astronomers to make fundamental tests of the nature of the fluctuations, which are enhanced by the astrophysics of the early universe.
The primordial fluctuations drove pressure variations through the fluid of matter and radiation that filled the early universe. These pressure variations are observable at the moment of last scattering. The largest detectable oscillations peaked for the first time at the horizon when the universe was 300 000 years old, but smaller fluctuations have undergone more oscillations and so peaked more frequently. The smaller the fluctuations, the less effective they are at trapping radiation. As radiation diffused out of the small-scale fluctuations the oscillations became progressively weaker. The net result is a series of decreasing peaks in the temperature fluctuations at angles that correspond to a half, a third and a quarter of the angle subtended by the horizon. The BOOMERANG, MAXIMA and DASI experiments provided evidence for the presence of up to three peaks. The latest results from the CBI and VSA experiments have confirmed this picture and, in the case of CBI, hinted at the existence of a fourth oscillation exactly as predicted by theory.
Peak viewing
The two latest experiments comprise an array of small telescopes that simulate a large dish but have a resolution that is determined by the size of the individual antennas. The VSA team – led by astronomers at Cambridge University in the UK – has made observations with an array of 14 antennas sited at an altitude of 2400 m in Tenerife. Each antenna is just 14 cm across. Meanwhile the CBI team – led by astronomers at the California Institute of Technology – operates an array of 13 antennas located some 5000 m above sea level in Chile’s Atacama desert. With a larger antenna diameter of 90 cm, the CBI can achieve a substantially higher angular resolution than the VSA.
Both sites in Tenerife and Chile are ideal for microwave astronomy because the atmospheric background is low at such high altitudes. This low background has enabled the experiments to match the exquisite temperature sensitivity of COBE over a small area of sky up to 100 square degrees across but with much higher angular resolution. Indeed the latest results from the CBI measure the temperature fluctuations to 1 part in 100 000 and harmonics to l = 3500.
Much of the detailed physics associated with the recombination of protons and electrons is expected to be visible in the cosmic microwave background. Astronomers can deduce a wide range of cosmological parameters from the location and strength of the peaks in the angular-power spectrum. For example, the locations of the peaks depend on the curvature of the universe and on the total density of matter and energy. If the universe is negatively curved, then the peaks would shift to smaller values of l. Meanwhile if the universe contains a smaller density of matter than predicted, then the peaks would be smaller.
The latest data from the VSA and the CBI, like previous observations, confirm that the geometry of the universe is Euclidean or flat and that the density of matter and energy is within 5% of the critical value predicted by Einstein’s general theory of relativity for a flat universe (see Boomerang backs flat universe Physics World June 2000 pp23-24). If the matter density were less than this value then it would expand forever.
Meanwhile the amount of normal or baryonic matter – that is all the matter made from protons and neutrons – that exists in the universe controls the relative strengths of the odd and even peaks, which are produced by compressions and rarefactions of the pressure waves. The reason is that baryons control the restoring force against the pressure waves. The results from the VSA and the CBI indicate that baryonic matter accounts for 5% of the critical density – precisely the value inferred from the synthesis of helium, deuterium and lithium in the first minutes of the Big Bang.
Cosmologists have known for decades that the motion of stars in the outermost parts of galaxies is due to the gravitational effect of non-luminous or “dark” matter. Since this dark matter does not couple to radiation it did not experience the pressure force or undergo oscillations. Yet dark matter does control the strength of the peaks in the power spectrum.
Astronomers can derive the matter density – that is the sum of the baryonic and dark-matter densities – from the absolute strength of the first peak. The latest experiments indicate that the matter density is 30 ± 20% of the critical density. This suggests that the missing two-thirds of the critical density might consist of an exotic form of “dark energy” that is quite distinct from dark matter in that it does not cluster under the influence of an attractive gravitational force.
Meanwhile the decline in the strength of the peaks observed by the CBI confirms a fundamental prediction of the standard cosmological theory, namely that we are witnessing the trace of acoustic fluctuations imprinted on the cosmic microwave background. It is these fluctuations that persisted and grew in the dark matter to eventually generate the galaxies and clusters of galaxies that we see today.
Scattering effects
The only surprise from the CBI results is the excess radiation near l = 3000, which is as large as the maximum expected contribution from galaxy clusters. The hot gas in clusters of galaxies can scatter the cosmic-microwave-background radiation and distort the background spectrum in a characteristic way. This phenomenon – known as the Sunyaev-Zeldovich effect – is relatively large in individual galaxy clusters. Indeed radio observations indicate that it reduces the temperature of the cosmic microwave background by about 1 mK. Now the CBI team has measured the effect for all galaxy clusters in the line of sight for the first time. The results show that the effect produces a temperature change of just 15 µK, which is much smaller than for an individual galaxy cluster.
Cosmologists are not entirely happy with the reported excess of radiation at large l. With such a relatively small area of sky surveyed by the CBI – only 40 square degrees – one has to be cautious about the statistical uncertainties inherent in sampling an inhomogeneous universe. Contributing radio sources may not have been completely removed. Indeed astronomers know that radio galaxies are often associated with galaxy clusters, so the Sunyaev-Zeldovich contribution may have been overestimated.
The true explanation of any possible excess requires astronomers to make observations at many different wavelengths over much larger areas of sky. These observations should also cover submillimetre wavelengths in order to view the scattered Sunyaev-Zeldovich photons. If the effect persists then either the Sunyaev-Zeldovich effect will be confirmed or else a more exotic explanation will be needed.
Such challenges could provide an exciting turn for cosmology that might take us beyond the concordance model that so far fits all of the observations of the cosmic microwave background and large-scale structure almost too perfectly.