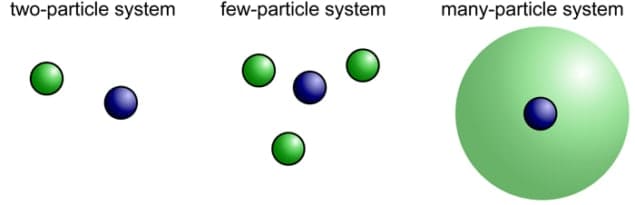
How big must an ensemble of particles be before the exact number of individual particles becomes inconsequential and the entire system can be described using many-body theories? This is an important question in condensed-matter physics and one that has proved difficult to answer. Now, a group of researchers in Germany has observed the transition from “few” to “many” in an experiment using ultracold fermionic atoms. The results could help in modelling simple, few-body systems and to study mesoscopic quantum-mechanical systems.
Complex interactions
Studying systems with many particles can be challenging: while the microscopic behaviour of each particle by itself might be easy to determine, their combined macroscopic behaviour as they interact can become very complicated. Indeed, for systems with anything more than three particles it is often impossible to find analytical or numerical solutions. One of the ways of getting round this problem is to assume that the system is made up of an infinite number of particles. By doing so, the variables of the system go from being discrete to continuous and this makes the system much easier to study. But knowing exactly when this transition from discrete to continuous occurs can be tricky. In the past, experimental studies had been done with systems such as helium droplets but the results were inconclusive.
A drop in the Fermi sea
Now, Selim Jochim, Andre Wenz and colleagues at the University of Heidelberg and the Max Planck Institute for Nuclear Physics, both in Germany, have observed this crossover by studying a quasi-1D system of ultracold atoms using identical fermionic lithium atoms. The experiments look at how an increasing number of lithium atoms interact with a single “impurity” atom – a fermion that is in a different spin state from the rest. The impurity is used to probe the behaviour of the majority atoms. By measuring the energy of the system the team found that the atoms exhibited characteristics of a many-body system – which is known as a Fermi sea – when as few as four majority atoms were present.
In the experiment, the team uses a system made up of six lithium atoms – one of which is the impurity. The majority atoms themselves do not interact with each other because of the Pauli exclusion principle that dictates that no two identical fermions (particles with half-integer spin) can occupy the same quantum state simultaneously. However, as the impurity has a different spin, it is not affected by the exclusion principle and so can interact with all of the majority particles at the same time. Tuning the interactions between the majority atoms and the impurity – using a phenomenon known as “magnetic Feshbach resonances” – allows the team to explore the crossover from “few” to “many” at different interaction strengths. By studying samples with different particle numbers, the energy of the system is determined as a function of the number of majority atoms, for varying strengths of interparticle interaction. Initially, the system consists of the impurity (blue) and several majority atoms (green) without any interactions (see figure). Then, the researchers introduce an interaction between the blue and green atoms by applying a magnetic field. The energy of the system is then determined by changing the spin state of the impurity atom using a radio-frequency pulse. And it is this shift that acts as a probe – if the majority atoms were not already acting as a many-body system, the transition of the impurity atom with the pulse would occur at a particular frequency. In the presence of the majority atoms showing their collective behaviour, the frequency of this transition energy is shifted. “For a many-body system we know at which frequency this transition should occur and by comparing this with the experimental results we can find out whether the system has already reached the many-body limit,” says Thomas Lompe, another member of the team who is now at the Massachusetts Institute of Technology in the US.
Designer system
The researchers found that for weak and intermediate interactions, four atoms is sufficient to warrant the use of a many-body theory to describe the behaviour of the system. Wenz told physicsworld.com that the result was surprising and was not theoretically clear when the researchers began their studies. He also says that their “designer” ultracold system itself was an achievement – the system is “tunable” in the sense that the researchers can control its size on a single-particle level while maintaining full control over interparticle interactions. Wenz explains that such intermediate mesoscopic states – when a system has neither too few nor too many particles – are important and difficult to model. Studying them in a regime where one can control both the number of particles and their interactions was a key achievement. Also, theirs was the first system of ultracold atoms where only a single impurity interacted with few majority atoms – previous experiments in 2D and 3D systems with thousands of impurity atoms embedded in even larger clouds of majority atoms had been carried out. In terms of 2D and 3D few-particle systems, Wenz speculates that the minimum number of particles necessary for collective behaviour would probably be higher, but possibly 10 particles might suffice.
Currently, the team is doing condensed-matter lattice simulations using multiple traps filled with ultracold atoms, where each trap represents a fixed lattice point. In the long term, Wenz suggests that such few-particle systems are useful to study superfluidity, to better understand the behaviour of nucleons in atomic nuclei as well as to test the descriptions of finite physical systems, such as the dopants in the tiny transistors that are currently being used in computers.
The research is published in Science.