The role of biophotons in the brain is a growing area of research in neurobiology – and where there are photons there might be quantum mechanics. Betony Adams and Francesco Petruccione explore this developing, and contentious, field of quantum biophysics
The light of the mind is blue, wrote the poet Sylvia Plath (“The Moon and the Yew Tree” 1961). But it seems it may actually be red.
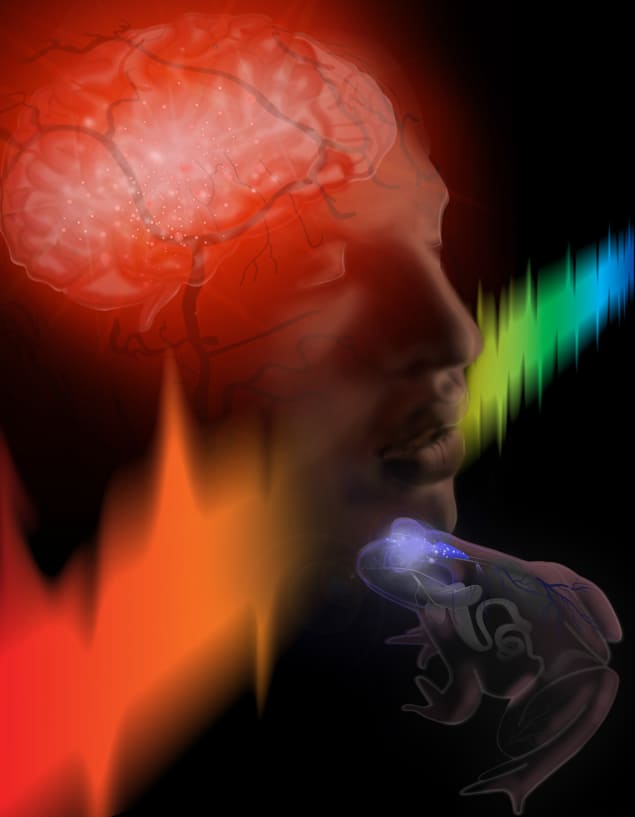
That’s because recent research suggests a link between intelligence and the frequency of biophotons in animals’ brains. In 2016 Zhuo Wang and colleagues at the South-Central University for Nationalities in China studied brain slices from various animals (bullfrog, mouse, chicken, pig, monkey and human) that had been excited by glutamate, an excitatory neurotransmitter. They found that increasing intelligence was associated with a shift in the biophoton’s frequency towards the red end of the spectrum (PNAS 113 8753).
Admittedly, it is unclear what the measure of intelligence actually is, and the study has drawn criticism for its lack of an explanatory mechanism; correlation, as the mantra goes, does not mean causation. However, the role of biophotons – spontaneous ultra-weak near-ultraviolet to near-infrared photons in biological systems – is a growing field of research in neurobiology.
Light has such symbolic resonance for humanity. It features in art, religion, literature and even in how we talk about knowledge – we speak of “enlightenment” and “seeing the light”, for example. It seems fitting, therefore, that it might play a physiological role as well. Just how light is involved in the signalling processes that constitute the central nervous system and its emergent property, consciousness, is still not clear. But inevitably, where there are photons, there might be quantum mechanics.
Photons, after all, are inextricably linked to the birth of quantum mechanics: Albert Einstein’s 1921 Nobel prize was awarded not for relativity or other discoveries, but for his explanation of the photoelectric effect. He theorized that light, which was conventionally accepted to behave as a continuous wave, might also be considered to propagate in discrete packages, or quanta, which we call photons. This, along with Max Planck’s understanding of blackbody radiation, Niels Bohr’s new model of the atom, Arthur Compton’s research into X-rays, and Louis de Broglie’s suggestion that matter has wave-like properties, ushered in the quantum age.
Quantum effects in the brain
While the weirdness of quantum theory has lent itself to some unhelpful pseudoscientific interpretations of consciousness, there has been resistance from scientists to yoke the two together. Just because both subjects are difficult to understand, does not mean that they necessarily inform each other. Despite this, the first detailed theory of quantum consciousness emerged in the 1990s from the Nobel-prize winning University of Oxford physicist Roger Penrose and anaesthesiologist Stuart Hameroff from the University of Arizona (Mathematics and Computers in Simulation 40 453). Their “orchestrated objective reduction” (Orch OR) theory has undergone a number of revisions since its inception (Physics of Life Reviews 11 39), but generally it posits that quantum computations in cellular structures known as microtubules have an effect on the firing of neurons and, by extension, consciousness.
The theory elicited a number of criticisms but perhaps the most damning followed from the fundamental tenets of quantum theory. A quantum system – which might refer for example to the dynamics of a photon – is a delicate thing. Conventionally, quantum effects are observed at low temperatures where this system is isolated from destructive interactions with its surrounding environment. This would seem to exempt quantum effects from playing any role in the mess and fuss of living systems. Biological systems, such as the brain, operate at physiological temperatures and are unavoidably bound to their environments. As calculated by physicist Max Tegmark at Princeton University in 2000, quantum effects would not survive long enough to have any influence on the much slower rates at which neurons fire (Phys. Rev. E 61 4194).
However, this objection has to some extent been mitigated by research done in the broader field of quantum biology. The application of quantum theory in a biological context has had most success with regards to photosynthesis but research on the avian compass, olfaction, enzymes and even DNA also suggest that quantum effects might be implicated more generally in the functioning of biological organisms.

Nerve constituents that are important to a discussion of quantum effects are the microtubules, which are formed from the polymerization of a protein known as tubulin, and the mitochondria, often described as the energy centres of the cell. Microtubules give structure to the cellular cytoskeleton and are necessary for cell division as well as the movement of motor proteins, a group of proteins that convert chemical to mechanical energy. The mitochondria use electron transport chains and proton gradients to create adenosine triphosphate (ATP), which powers biological processes. They are also the proposed primary site of biophoton production. (Illustration by Angela Illing. Reproduced from AVS Quantum Sci. 2 022901, with the permission of the American Vacuum Society)
In a trivial sense all biology is quantum mechanical just as all matter is quantum mechanical – it is made up of atoms and thus subject to the physical laws of atomic structure first formalized by Bohr at the beginning of the 20th century. The focus of quantum biology, however, is on key quantum effects – those quantum phenomena that seem to defy our classical imaginations, such as superposition states, coherence, tunnelling and entanglement (see box “Quantum phenomena”).
If this is the what of quantum effects in the brain, the where is more straightforward. The brain is made up of nerve cells – elongated cells consisting of a cell body, dendrites and axon (figure 1). Put simplistically, information is passed to and from the brain by the firing or not firing of neurons, a process determined by a nerve cell’s electrochemical potential. This potential depends on the spread of charged ions across the cell membrane, making either side of the membrane more or less positive. In order for a nerve to fire, its resting potential must be increased to the requisite threshold potential. How this signal then passes from one cell to the next is still a matter of debate, but the accepted theory is that this neural communication is managed by chemicals known as neurotransmitters released into the synaptic cleft, which then bind to receptors of the next nerve cell, thereby altering its electrochemical gradient and causing neural activation.
Altered states of consciousness
What better way to study consciousness than by looking at it in altered states – specifically the chemicals that achieve this, such as general anaesthetics. “The only thing we are sure about consciousness, is that it is soluble in chloroform,” said quantum biologist Luca Turin of the Alexander Fleming Biomedical Research Centre in Greece in 2014 (EMBO Reports 15 1113). Turin noted that chemicals with anaesthetic capabilities have chemical and structural properties that are very different from each other, leading him to focus on the similar physics that these substances might share. Anaesthetics can bind to various cytoplasmic and membrane proteins. He proposed that anaesthetics facilitate electron currents in these proteins and that this might be demonstrated by looking at changes in quantum spin, where spin describes the magnetic properties of quantum particles such as electrons. What he found was that under the influence of xenon, the simplest of all the anaesthetics, fruit flies showed an increase in electron spin as measured through the use of electron spin resonance (though the origin of the signal is still debatable).
The involvement of anaesthetics in the electronic properties of biological systems is not a completely new theory, having been outlined by Hameroff in addition to Orch OR. What is new is the progress made in understanding how quantum effects might contribute to electronic transfer processes in biological systems. In photosynthesis, there is some evidence that the movement of energy through the structures that constitute the photosynthetic network exploits quantum effects such as coherence (see April 2018 feature “Is photosynthesis quantum-ish?“). Specifically, the structures that seem to allow this coherent transfer are chromophores, the parts of a molecule that give it its colour. Research suggests that instead of moving between the discrete energy levels of an arrangement of chromophores, energy can be spread out or delocalized across more than one chromophore at a time.
What is interesting in the context of quantum consciousness is that nerve cells contain structures such as microtubules and mitochondria that might support coherent energy transfer in a manner similar to that in photosynthesis. Microtubules form part of the cytoskeleton of eukaryotic cells (those with a nucleus enclosed in an envelope, found in plants and animals) and some prokaryotic cells (those with no nucleus envelope, which archaea and bacteria are made of). They provide shape and structure, and are instrumental in cell division as well as the movement of motor proteins. They are made up of polymers of tubulin proteins and within these are chromophores similar to those found in photosynthetic networks. Chromophores are also found in mitochondria, the power stations of the cell. This had led some researchers to suggest that anaesthetics work by disrupting coherent energy processes and in turn disrupting consciousness.
Anaesthetics are not the only chemicals implicated in altered states of consciousness. It is generally accepted that disruptions in the action of neurotransmitters, the molecules by which neurons communicate, contribute to a variety of mental illnesses. Antidepressants, for example, are thought to work by increasing neurotransmitters such as serotonin, the poster-chemical for happiness. However, the exact mechanism of neurotransmitter action is still not perfectly understood. Conventional theory has it that they bind to membrane receptors on nerve cells through a lock-and-key mechanism, where the shape of a particular neurotransmitter matches the shape of the appropriate receptor. The lock-and key mechanism is associated with a number of biological functions, one of which is olfaction (your sense of smell).
However, an alternative theory of olfaction suggests that it may use principles of vibration-assisted quantum tunnelling rather than relying on molecular shape. Recently this theory has been applied to the action of neurotransmitters as well. Vibration-assisted tunnelling is when the energy of a molecule’s movement matches the energy necessary for an electron to tunnel through a potential barrier. In this sense the vibration of a particular neurotransmitter would be recognized by its specific receptor. Using mathematical and computational modelling, researchers tested this by looking at isotopes of different neurochemicals such as serotonin, histamine and adenosine (a review of these studies can be found in AVS Quantum Sci. 2 022901). As their mass changes but their shape remains the same, their vibrational frequencies are altered. The researchers were looking to see whether neurotransmitter isotopes had differing effects, thus disqualifying the lock-and-key mechanism, which depends on shape, and supporting the possibility of vibration assisted tunnelling. Although theoretical results look promising the theory has yet to be firmly supported experimentally.
Quantum phenomena
In quantum biology, the quantum effects of superposition, coherence and decoherence, tunnelling, and entanglement play an important role.
Mathematically, a physical system – for instance an atom or photon – is described by a quantum state that contains all the information about it. Superposition is a property of the quantum world that allows a physical system to exist in two or more quantum states, until a measurement is made on it. The non-intuitive phenomenon prompted Erwin Schrödinger’s famously ubiquitous thought experiment where a cat in a box is simultaneously dead and alive until an observer looks in the box. Quantum coherence quantifies this relationship of states in a superposition. And its counterpart, decoherence, describes the loss of such quantum effects.
Quantum tunnelling, meanwhile, involves a particle passing through an energy barrier despite lacking the energy required to overcome the barrier, as would be defined by classical physics. The phenomenon is not fully understood theoretically, yet it underpins practical technologies ranging from scanning tunnelling microscopy to flash memories.
Finally, quantum entanglement allows two particles, such as photons or electrons, to have a much closer relationship than is predicted by classical physics. Over the years, it has played a central role in quantum technologies such as quantum cryptography, quantum teleportation and networks for distributing quantum information. Over the past decade, physicists have been able to transmit pairs of entangled photons over increasing distances, both in the air and along optical fibres.
The brain’s compass
A number of animals are able to sense Earth’s magnetic field, but exactly how they accomplish this is still an open question. Birds, it has been hypothesized, use quantum effects to accomplish their feats of navigation. This quantum compass is called the radical pair mechanism and it relies on the interaction of electron spin with the geomagnetic field. A radical pair is a pair of electrons whose spins are correlated, existing in a superposition of two different states. The ratio of these states is determined by the magnetic field, resulting in a different chemical signature for different alignments in this field. This spin-dependent compass is thought to be located in molecules known as cryptochromes, which are activated by blue light from environmental cues. Until very recently there was no strong evidence that humans had a magnetic sense. However, a new experiment by Kwon-Seok Chae and team at Kyungpook National University in Korea shows, incredibly, that starved humans can sense the geomagnetic field to orient themselves towards the remembered location of food, an orientation that appears to be blue-light dependent (PLOS One 14 e0211826).
It has also been shown by Connie Wang from the California Institute of Technology, US, and colleagues that changes to the strength of Earth’s magnetic field cause changes in alpha brain waves – oscillations in the neural activity of the brain in the frequency range 8–12 Hz – in human subjects (eNeuro 6 ENEURO.0483-18.2019). However, it is uncertain whether this effect uses a similar quantum mechanism to the avian compass – in fact, the researchers suggest quite the opposite, that ferromagnetism is responsible for the effect.
In separate studies, changes in alpha waves have been associated with fluctuations in the production of biophotons, measured indirectly by fluctuations in reactive oxygen species, which play a role in cellular communication but are also responsible for numerous bodily problems. They are implicated in ageing, disease and depression and are the reason that antioxidants are so widely touted as being beneficial to health. What is interesting is that studies have shown how magnetic field mediated changes in the spin dynamics of the radical pair mechanism lead to increased reactive oxygen species. It is conceivable, though as yet contentious, that humans use the radical pair mechanism in essential cellular functioning. Exactly what this entails is less clear. It could potentially offer a means to understand the apparent physiological and psychological effects of geomagnetic storms, one of which appears to be increased rates of suicide (Proc. R. Soc. B 279 2081).
Neural entanglement
Spin dynamics, the behaviour of quantum particles in a magnetic field, is also at the heart of another theory that suggests that quantum effects play a role in cognition. In this case, however, the spins in question belong to nuclei rather than electrons. Nuclei can have particularly long coherence lifetimes, meaning that their quantum effects persist over timescales long enough to play a role in neural firing and even, possibly, the function of memory.

This notion led physicist Matthew Fisher, from the University of California, Santa Barbara, to suggest that spin-entangled molecules known as Posner molecules might lead to nerves firing in a correlated fashion. This happens through a number of steps. Cellular processes run on energy that is provided by the chemical compound adenosine triphosphate (ATP). When this compound is broken down, it releases phosphates, which are made up of phosphorus (spin-half nuclei) and oxygen (zero nuclear spin). Fisher contends that the spins of the phosphorus nuclei are entangled and that, furthermore, if this quantum entanglement can somehow be isolated from other quantum interactions it might last long enough to have an effect on cognition processes (Annals of Physics 362 593).
He suggests that the phosphates form Posner molecules by binding with spin-zero calcium ions, which act as an effective screen from external interactions. Entangled Posner molecules are then taken up into neurons, bind and release calcium ions, triggering entangled neural activation. Fisher uses this model to suggest why lithium is successful in treating bipolar disorder. Should lithium replace the central calcium ion in a Posner molecule then the non-zero spin of the lithium ion could contribute to decoherence and have a knock-on effect on neural activation.
What is perhaps more surprising with regards to lithium is that different isotopes have been shown to have differing effects on the mothering behaviour of rats. A similar phenomenon has recently been recorded in the action of xenon, an anaesthetic. Na Li and colleagues at Huazhong University of Science and Technology in Wuhan, China, found that differing isotopes of xenon cause differing levels of unconsciousness (Anesthesiology 129 271). This seems extraordinary, that changing something as small as the spin of a nucleus might result in macroscopic changes on the level of something as complex as the mothering instinct or, indeed, consciousness itself.
But what’s the use?
While the possibility of quantum effects in the brain is intrinsically fascinating, it might also contribute to the ways in which we treat the brain and disorders related to the brain.
Unravelling exactly how neurotransmitters bind to receptors would contribute to understanding G-protein coupled receptors, such as neural and olfactory receptors, which are one of the primary targets of most pharmaceutical intervention. But more than this, identifying how quantum effects might play out in the brain could offer a completely new way of imagining medical intervention beyond the purely chemical. For example, it could help refine and enhance electroconvulsive therapy (the transcranial application of electric currents) and the less well established but also less invasive method of transcranial magnetic stimulation (the use of magnetic fields to stimulate parts of the brain) as treatments for depression.

Is photosynthesis quantum-ish?
Deciphering the role of light could also be beneficial as a number of recent studies have shown it to have a range of physiological effects. Researchers have found that shrimp exposed to excess serotonin-based antidepressants from human contamination were more likely to seek out light, a result that led to increased predation (Aquatic Toxicology 99 397). While detrimental to the shrimp, this might tell us about how much of our own physiology is responsive to light, and to what extent light could be pharmaceutically useful.
In another recent study, quantum dots – semiconducting nanoparticles capable of producing light – were successfully used to undo the protein clumping linked to Parkinson’s and Alzheimer’s disease (Nature Nanotechnology 13 812). Meanwhile, declining eyesight has been shown to improve through the amelioration of mitochondrial damage by red light treatment (The Journals of Gerontology: Series A 75 e49). Photobiomodulation, the application of red or near-infrared laser light, has also shown promise in treating various brain disorders, as well as improving attention, memory and learning (BBA Clinical 6 113).
An illumination in every sense of the word, it appears that there may be more than metaphor to the action of becoming enlightened.