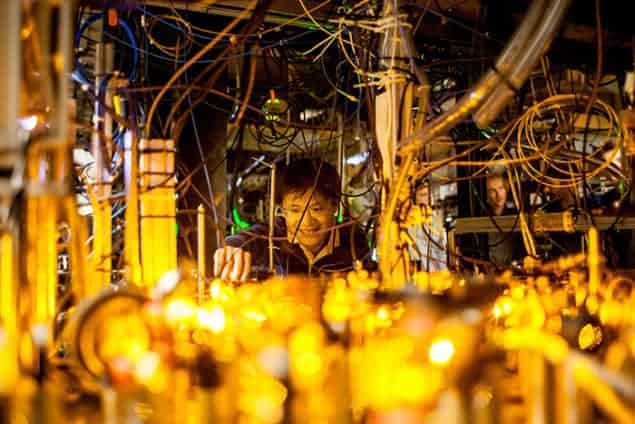
A microscope that can see up to 1000 individual fermionic atoms has been developed by a team of physicists in the US. Using two laser beams, the research team traps a cloud of potassium atoms in an optical lattice, cools the atoms and then simultaneously images them. The new technique allows researchers to clearly resolve single fermions, directly observe their magnetic interactions and even detect entanglement within the ensemble.
Fermions are particles that have half-integer spin, and therefore are constrained by the Pauli exclusion principle, which dictates that no two identical fermions can occupy the same quantum state simultaneously. Fermions include many elementary particles – quarks, electrons, protons and neutrons – as well as atoms consisting of an odd number of these elementary particles. As a result, the collective behaviour of fermions is responsible for the structure of the elements in the periodic table, high-temperature superconductors, colossal magnetoresistance materials, the properties of nuclear matter and much more. Despite their importance, however, we still do not have a complete picture of strongly interacting systems of fermionic particles because they are notoriously difficult to image and study.
Researchers have been studying bosons – particles that have integer spin and can occupy the same quantum state – by cooling clouds of bosonic atoms down to temperatures near absolute zero to form a Bose–Einstein condensate and then studying their interactions. But doing the same with fermions is no mean feat – the exclusion principle does not allow two fermions to be in exactly the same state. Therefore, as more fermions are added to a system, each succesive one comes in at an increasingly higher energy, making the system very tricky to cool. Furthermore, ultracold atoms are easily perturbed by just the light from a single photon, which makes it difficult to confine atoms for long enough to obtain a clear image.
Supercool light
To get round these problems, Lawrence Cheuk, Martin Zwierlein and colleagues at the Massachusetts Institute of Technology have developed a microscopy technique that involves imaging the atoms with the same light that cools them. The fermions are first cooled to a temperature of just above absolute zero using standard methods, including laser cooling, magnetic trapping and evaporative cooling of the gas, until the temperature of all of the atoms is just above absolute zero. At this point the atoms settle into the wells of an optical lattice, thereby stopping any contact between neighbouring fermions and preventing them from interacting with each other. The optical lattice is located just 7 μm from the microscope’s imaging lens, and is made of criss-crossing laser beams that form an “egg carton” structure with a fermion trapped in each well.
The atoms are then cooled even more by using two lasers, each at a different wavelength. This method makes use of Raman transitions: an atom absorbs one photon, is immediately stimulated to emit another and so drops down one vibrational level in the process. The location of each of the atoms is identified by the stimulated photon that it emits as it cools. These photons are captured by the microscope lens above the lattice, and this allows the team to detect the fermion’s exact position within the lattice to an accuracy better than the wavelength of the light.
Using this method, Zwierlein and colleagues were able to cool and image more than 95% of the atoms in a potassium-40 gas cloud. The team was surprised to find that the fermions remained cold even after the imaging was complete. “That means I know where they are, and I can maybe move them around with a little tweezer to any location, and arrange them in any pattern I’d like,” says Zwierlein. To make sure that their experiment did not suffer any light-assisted losses, the researchers looked at how the atoms move around between successive images, and at the statistics of how the atoms are distributed around the lattice. The team found that it was not losing a significant number of atoms.
Cold-atom toolbox
Chad Orzel, a physicist at Union College in the US who was not involved in the work, is impressed with the research because it opens up the possibility of using fermionic atoms to create a wider range of condensed-matter analogues. “If you look at the behaviour of bosons in an optical lattice, that’s analogous to the behaviour of superconductors, where electrons have paired up to act like bosons. But a system of fermions in a lattice is more analogous to a normal conductor, where the electrons are subject to Pauli exclusion, and you can see other fun behaviours that way.” He adds that with fermionic systems, “you can also think about using light fields to manipulate the interactions between atoms in interesting ways, and watch how particles move around”. Orzel told physicsworld.com that Zwierlein’s work is a nice addition to the cold-atom experimental toolbox. “Because the atoms are out in the open and directly imaged, you have all sorts of freedom to change parameters without needing to make whole new samples,” he adds.
The research is published in Physical Review Letters.