As we celebrate 50 years of the laser, a milestone looms in the world of laser fusion. Mike Dunne describes how achieving ignition – fusion’s break-even point – with the world’s largest laser will transform the search for abundant, carbon-free electricity
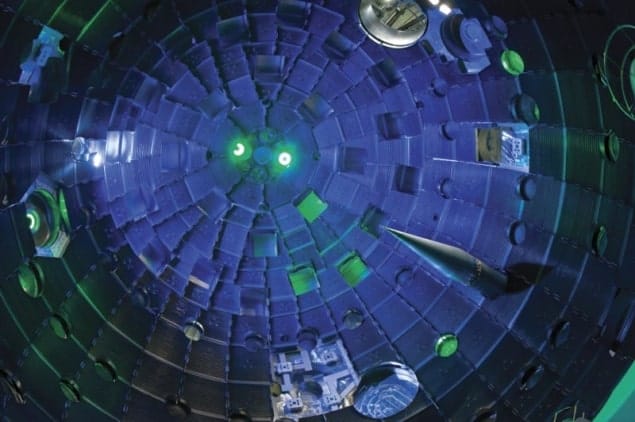
Three days after Theodore Maiman demonstrated the first ruby laser at his laboratory in Malibu, California, in May 1960, a scientist a few miles away at the Lawrence Livermore National Laboratory came up with an idea for using lasers to harness the power source of the stars. Although details of Maiman’s device would not emerge for several weeks, scientists already knew that a laser’s ability to concentrate energy in time and space would be unprecedented. Might it be possible, the Livermore scientist wondered, to use lasers to fuse small atoms together to create a heavier, more stable atom – releasing huge amounts of energy in the process?
Thanks to the levels of secrecy prevalent at the time concerning atomic matters, it would be another 12 years before the scientist in question, John Nuckolls, articulated his ideas about laser fusion for the broader scientific community. Writing in Nature, Nuckolls and his colleagues explained that in order for their scheme to work, a large-scale laser had to be built – one that could compress and heat the fusion fuel to a temperature of 108 K and densities 1000 times that of liquids, conditions that surpass even those found at the centre of the Sun.
Nuckolls’ team predicted that a laser with an energy of 1 kJ and a pulse length of a few nanoseconds would be sufficient to initiate the process, although a much larger laser (a few megajoules, it was estimated) would be required to produce a substantial energy output. Scientific excitement over this idea – coupled with a succession of energy crises in the 1970s and 1980s – led to the construction of a series of increasingly large lasers to test the concept. Unfortunately, these experiments proved that the journey would be much harder than predicted: the threshold itself was likely at the megajoule level, thanks to the need to overcome a range of instabilities that hampered efforts to couple laser energy to the fuel and then compress it to the required densities.
Yet after years of intermittent successes and setbacks, we are finally entering a truly exciting period in the world of laser fusion. The past decade has seen unprecedented sums of money invested in the field, with the principal aim of demonstrating, once and for all, that the science of laser fusion really works. The recently completed US National Ignition Facility (NIF), located at the same lab where Nuckolls had his big idea 50 years ago, is among the most tangible results of this effort (see “The National Ignition Facility”). And a little over a year after NIF officially opened, scientists there are now on the brink of a breakthrough: crossing the required threshold for the instigation of a self-sustaining fusion reaction, leading to a net release of energy for the first time.
The achievement of this 50-year-old goal – known technically as “ignition” – will be a game-changing event that will propel laser fusion from an elusive phenomenon of physics to a predictable, controllable, technological process ready to address one of society’s most profound challenges: finding an enduring, safe and environmentally sustainable source of energy. The NIF plan is to ensure that this milestone is reached within the next two years.
Making a star in the lab
The history of fusion can be traced back to 1920, when Francis William Aston discovered that four separate hydrogen nuclei are heavier than a single helium nucleus. This occurs because the stability of helium leads to a lower overall rest mass. On the basis of this work, another British scientist, Arthur Eddington, proposed that the Sun could get its energy from converting hy_drogen nuclei into helium nuclei, releasing just less than 1% of the mass as energy, according to Einstein’s famous equation E = mc2. Then, in 1939, Hans Bethe distilled these facts into a quantitative theory of energy production in stars, which eventually won him the 1968 Nobel Prize for Physics.
Although the Sun and other stars generate fusion by using their gravitational energy to compress hydrogen (and subsequently heavier elements), for any terrestrial effort it makes more sense to use a fuel source composed of deuterium and tritium. These isotopes of hydrogen contain one and two neutrons, respectively (see “Getting it together”). They have the highest cross-section for fusion since they have low charge (just a single proton each) and the proton and neutron(s) are not very tightly bound. In the basic fusion reaction, deuterium (D) and tritium (T) combine to form helium and a very energetic neutron: 2D + 3T → 4He (3.5 MeV) + n (14.1 MeV)
In order for this reaction to take place, the particles need to be moving at very high velocities to overcome the Coulomb barrier, since the positive ions experience an increasingly strong repulsive force as they get closer and closer together. This means that the fuel needs to be heated to an incredible 108 K. Under these conditions, electrons are stripped from their parent nuclei, turning the fuel into a plasma.
The need to create high-temperature plasmas for fusion to occur explains why fusion is not a process we encounter in everyday life on Earth, and why it is so incredibly difficult to harness as a net source of power. On a positive note, this does introduce one major benefit: unlike nuclear fission, which can lead to an uncontrolled “chain reaction”, the fusion process is inherently safe since the fuel “wants” to be inert, and thus loses energy at any opportunity. And thanks to the stars, we know categorically that fusion works – we just need to find an alternative to the Sun’s use of gravity to provide the heating and confinement of our fuel.
There are two principal routes to achieving confinement: we can either hold the plasma in a magnetic field while heating it using radio waves or particle beams; or we can compress it to unprecedented densities using lasers. The first approach is being pursued through the ITER magnetic-confinement fusion experiment currently being built in Cadarache, France, while the latter is being studied at handful of labs – including NIF – using some of the world’s largest lasers.
How laser fusion works
The laser route to fusion neatly combines two of Einstein’s most famous contributions to science: his explanation of stimulated emission; and his quantification of the equivalence of mass and energy. The basic approach is a repetitively cycled system in which ball-bearing-sized pellets of deuterium–tritium fuel (see “On target”) are injected into the centre of a large, empty chamber. A number of powerful laser beams are used to compress the fuel to densities of 1000 g cm–3, or about 100 times the density of lead, for a few millionths of a millionth of a second (10–12 s). Of course, this high-density fuel will subsequently blow apart – but not instantaneously. It will persist at high densities on a timescale determined by its inertia and characterized by the time taken for a sound wave to propagate across the imploded assembly. This “self-confinement” phenomenon has led to the process being called “inertial-confinement fusion”, and it gives the system sufficient time to allow a substantial fraction of the fuel (typically 30%) to be converted to helium and a neutron.
The first fusion reaction produces a helium ion that deposits its energy in the neighbouring fuel, thus allowing the high temperatures to be maintained and the fusion reaction to propagate through the fuel. The high-energy neutron, however, escapes, since it interacts only weakly with the charged plasma. The neutron’s energy is therefore carried into a thick “blanket” of material surrounding the interaction chamber, heating the blanket to about 1000 K. In a fusion power plant, the process would be repeated about 10 times per second, and the heat would be used to drive an advanced gas-turbine cycle, thereby generating electricity.
The physics underpinning laser fusion is actually quite well understood. Moreover, thanks to a series of experiments performed by UK and then US scientists in the 1980s (see Physics World March p23, print edition only), we know that ignition and energy production can be attained here on Earth if we have a sufficiently powerful driver. These experiments, which used the X-ray output of an exploding thermonuclear bomb to implode the pellets, can be viewed as the ultimate “swords into plough shares” demonstration. What remains is to prove that a laser can be used as the driving source, and to demonstrate that the emitted fusion energy can be harnessed at a level compatible with a full-scale power plant.
The deuterium in the fuel pellet is sourced from water, which naturally contains about one molecule of D2O for every 6000 molecules of H2O. The tritium, in contrast, must be manufactured in situ by bombarding lithium-6 atoms with neutrons, thereby transmuting the lithium into tritium and helium. Here, we can use a neat trick: if we construct the blanket surrounding the fuel pellet with lithium-6, we can use the neutrons produced in the fusion reaction to generate more tritium (as well as producing the heat for the electricity turbine). In practice, it is a little more complicated than this, because we have to ensure that there are enough excess neutrons to create a closed fuel cycle; however, this can be achieved by adding other materials (principally lithium-7, beryllium or lead) to the blanket.
On the laser side, Nuckolls’ original predictions that a relatively small-scale laser would be sufficient to create the required conditions turned out to be correct only if there is freedom to drive the implosion at an arbitrarily high velocity. This is not possible due to various unstable, nonlinear processes in which the laser can set off electron or ion “waves” in the plasma, or cause the imploding fuel to break up prior to reaching high compression. For example, when high-intensity lasers heat matter, they can resonantly drive an oscillation in the plasma, thus causing the light to scatter off the plasma wave and preventing the fuel from absorbing it efficiently. If the laser intensity is too low, however, then the pellet implosion is driven at such a low velocity that any imperfections arising from surface roughness or laser non-uniformities seed the growth of hydrodynamic instabilities, leading to total break up of the imploding shell prior to full compression.
It has taken many decades to adequately understand these processes, and their existence has meant that a laser roughly 1000 times the scale originally envisaged by Nuckolls has to be used. The lasers at NIF – which have been performing remarkably well in their initial phase of operation – are designed to mitigate the growth of these plasma and hydrodynamic instabilities. Much attention has been paid to ensuring a sufficiently “smooth” laser beam, with control over its temporal profile to allow quasi-isentropic compression of the fuel by launching a series of precisely tailored shocks.
From fusion to electricity
Fusion physicists are so confident that NIF will be able to “ignite” a self-sustaining fusion reaction that attention is now turning to the endgame. The next problem is how to best harness the emitted neutrons in a manner compatible with a robust, commercially viable power plant. Such a plant would operate conceptually like a car engine, with three key stages.
In the first step, fuel – in the form of a ball-bearing-sized pellet of frozen hydrogen isotopes, held at temperatures of about 18 K – is injected into a multi-metre-diameter vacuum chamber. Next, a laser “piston” compresses the fuel by heating the outer surface of the pellet to create a hot, spherically expanding gas. In order to conserve momentum, the rest of the pellet is forced to move rapidly inwards at velocities of more than 105 m s–1. The degree of compression achieved in this process is similar to squashing a basketball down to the size of a pea.
In advanced schemes – analogous to a petrol engine – a separate laser is then used as a “spark plug” to ignite the fuel at the instant of maximum compression. Adding in this extra laser could lead to a more efficient (higher gain) system, but it is not an essential requirement: if we compress the fuel enough, the compression alone will generate enough heat to create a hot “spark” at the centre of the imploding fuel. When the temperature is high enough, and enough mass has been imploded to an appropriately high density, fusion is initiated in a self-sustaining manner. The helium nucleus from one reaction heats the neighbouring fuel, while the neutron escapes to heat the external blanket to generate electricity.
The final step occurs when the spent fuel is exhausted out of the chamber. At this point the cycle repeats. In a car engine, the fuel cycle is repeated about 50–100 times per second. The repetition rate for laser fusion is lower: 10 times a second would be enough to produce electricity on the gigawatt scale, comparable to the largest coal, gas or fission power stations. However, that rate is simply not possible with NIF, which fires only once every few hours. New technology is needed to convert the scientific demonstration on NIF into a constantly cycling system that can generate electricity.
One project that aims to bridge the gap between achieving ignition and building a practical fusion power plant is the High Power laser Energy Research facility, or HiPER. Led by the UK and involving a 10-nation consortium of researchers and funding bodies, HiPER’s goal is to demonstrate the 10 Hz level of performance of all the component technologies for power-plant-scale operation within the next 10 years. To do this, we hope to draw on innovations that are taking place elsewhere in laser science, including the high-repetition-rate technology used in the welding and machining industry, and several ongoing high-power-laser research projects. One example of the latter is the Extreme Light Infrastructure (ELI) project, a €750m effort led by the Czech Republic, Hungary and Romania (see pp12–13, print edition only) that seeks to create laser pulses with peak powers of up to a few hundred petawatts (about 1017 W) using the same type of diode-pumped laser technology that HiPER will require (see “Laser technology for fusion power”).
Over the past few decades, lasers have developed at an incredibly fast pace, allowing fusion researchers to take advantage of rapid increases in power and efficiency. Using lasers also allows us to adopt a modular, maintainable and easily upgraded approach to power-plant design during HiPER’s second phase, in which we plan to build a facility that combines the scientific demonstration of ignition at NIF with high-repetition-rate laser technology. This modular strategy should reduce the timescale for construction, increase power-plant availability throughout its life, and ensure that we find the most cost-efficient solution.
At the same time as Europe is devoting resources to HiPER, US scientists are planning a similar journey with the aptly named LIFE project (Laser Inertial Fusion Engine). Led by the scientists who worked on NIF, this project has the same goal as HiPER: to demonstrate the required high-repetition-rate technology, integrated into a power-plant-scale facility. Scientists in Japan, meanwhile, have well-defined plans for demonstrating the “petrol engine” approach to power generation described above. Thanks to these efforts, it is looking increasingly likely that reaching ignition at NIF will remove the question of whether laser-fusion power will be achieved, to replace it with the more political question of who is likely to deliver the first working power plant.
Towards a working power plant
The achievement of ignition at NIF will provide the ultimate verification of the scientific basis of laser-fusion energy, marking the culmination of 50 years’ effort. Yet the second milestone – a working fusion power plant – is the real goal, motivated by the demand for a sustainable, low-carbon economy. As we have already seen, the principal ingredients in fusion are deuterium, which is found in water, and lithium, which occurs naturally in igneous rocks and some types of clay, as well as in seawater. The Earth contains enough of both ingredients to last for millennia. In fact, based on current rates of electricity consumption in the UK, just one bathtub of water and the lithium from two laptop batteries would provide enough electricity for an individual’s entire lifetime.
Furthermore, fusion produces no greenhouse-gas emissions and has a low environmental impact over the life-cycle of a plant. The chief waste product is inert helium gas, and the residual radioactivity at the plant itself should be manageable using conventional decommissioning techniques over a period of 100 years. Fusion plants will have power outputs of as much as 1–2 GW, making them ideally suited as large, central facilities on the existing electricity-grid infrastructure. Other benefits include the high-temperature environment of the blanket, which could be used to generate hydrogen for fuel cells or even to desalinate water. These wider applications, as much as their electricity output, may be the crucial factor that will determine the commercial viability of early fusion power plants, and thus the timescale for delivery of the first generation of facilities.
In the meantime, laser facilities used in the pursuit of fusion can also be exploited for pure research. The topics range from studies of astrophysical processes such as nucleosynthesis, cosmic-ray generation, proto-stellar jets and planetary-nebulae formation, to the research into the cores of gas-giant planets and the origins of the Earth’s magnetic field. The lasers could also underpin a host of fundamental studies in areas as diverse as atomic physics, nuclear science, turbulence and the creation of macroscopic quantities of relativistic matter.
Perhaps just as importantly, the component technologies used in fusion research – not least the highly efficient, high-power lasers themselves – open up a wide range of spin-off opportunities. These range from security screening for nuclear materials at ports and the production of medical radioisotopes to the treatment of deep-seated tumours via particle-beam therapy, the processing of materials for the aerospace industry and even the development of next-generation light sources.
Pursuing a future energy source based on lasers still faces huge technological challenges in advanced materials, micro-scale engineering, laser technology and integrated power-plant systems. But the wider market for the high peak-power, high average-power laser systems allows the fusion field to build from a well-developed industrial base, and to borrow advances from other projects to accelerate the timescale to delivery. We have been waiting 50 years for the scientific proof that controlled fusion works. Now that this proof is almost upon us, we need to make sure we capitalize on it to ensure that we do not have to wait a further 50 years to see it used.