Half a century after the historic Apollo 11 landing on 20 July 1969, Robert P Crease explains why the mission’s lunar laser ranging experiment is alive and well – and still encountering new frontiers
The Moon has serenely and inexorably circled the Earth for millennia. Humans have regarded this glinting, mysterious object as both a source of wonder and as a fundamental landmark in the universe. The ancient Greeks even believed there was a sphere carrying the Moon that was the demarcation between our ever-changing, sublunary world and the incorruptible, unchanging and regularly moving heavens.
However, our relationship with our nearest neighbour has never altered so fast as it did after US President John F Kennedy spoke to the US Congress on Thursday 25 May 1961, a speech in which he committed his country to landing a human on the Moon by the end of the decade. It was a bold vision that was fulfilled on Sunday 20 July 1969 when Apollo 11’s Eagle lander touched down in the Sea of Tranquillity and Neil Armstrong took those first, faltering footsteps on the Moon.
The landing was a remarkable feat, watched by an estimated TV audience of 600 million, with Apollo 11 seeming to encapsulate the Zeitgeist of the age. Any scientific and technological challenge, no matter how grand, was surmountable if enough resources and expertise were devoted to it. Space was the new frontier – and science and technology would change the world for the better. Even today, the image of Buzz Aldrin in a white spacesuit, with a piercing black shadow cast on the lunar floor and Armstrong visible in the visor, remains iconic.
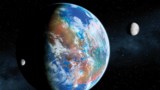
What’s in a moon?
But the Apollo 11 mission did not just push human technology, engineering and endeavour to the limit. It also had science on board (see “Giant leaps for knowledge”). In particular, there was an ambitious experiment to install mirrors on the lunar surface. If light emitted by Earth-bound telescopes could be made to bounce off those surfaces, it would reveal the precise distance between the Earth and Moon. Previous studies using radar had shown the distance to be about 385,000 km (depending on the Moon’s orbital position) with an error bar of ±1 km.
Designed to improve on that measurement of the Earth–Moon distance, the Apollo 11 experiment duly fulfilled its goal. In fact, it became known as NASA’s most cost-effective experiment. But why, half a century on, are the results still challenging physicists?
Eagle’s egg
The roots of the new method for measuring the distance to the Moon originated in the late 1950s when Princeton University physicist Robert H Dicke, along with his collaborators and students, imagined mounting mirrors onto satellites. If they could bounce light off those on-board mirrors, researchers could not only precisely determine the orbital characteristics of the craft, but also obtain various fundamental scientific insights. These included tests of general relativity, and measurements of whether the gravitational constant G is not fixed but changes over time, which would be manifested by variations in the satellites’ orbits.
Shortly after Kennedy’s 1961 speech, Dicke’s students hatched a scheme to have spacecraft plant arrays of mirrors on the Moon. With the laser having been invented the year before (1960), they figured it might be possible to direct laser light off such an array and have the return light be detected by an Earth-based telescope. The time taken for the light to do the round trip – the “range” – would measure twice the distance between the telescope on the Earth and the array on the Moon. That in turn could be used to test general relativity and search for possible variations in G.
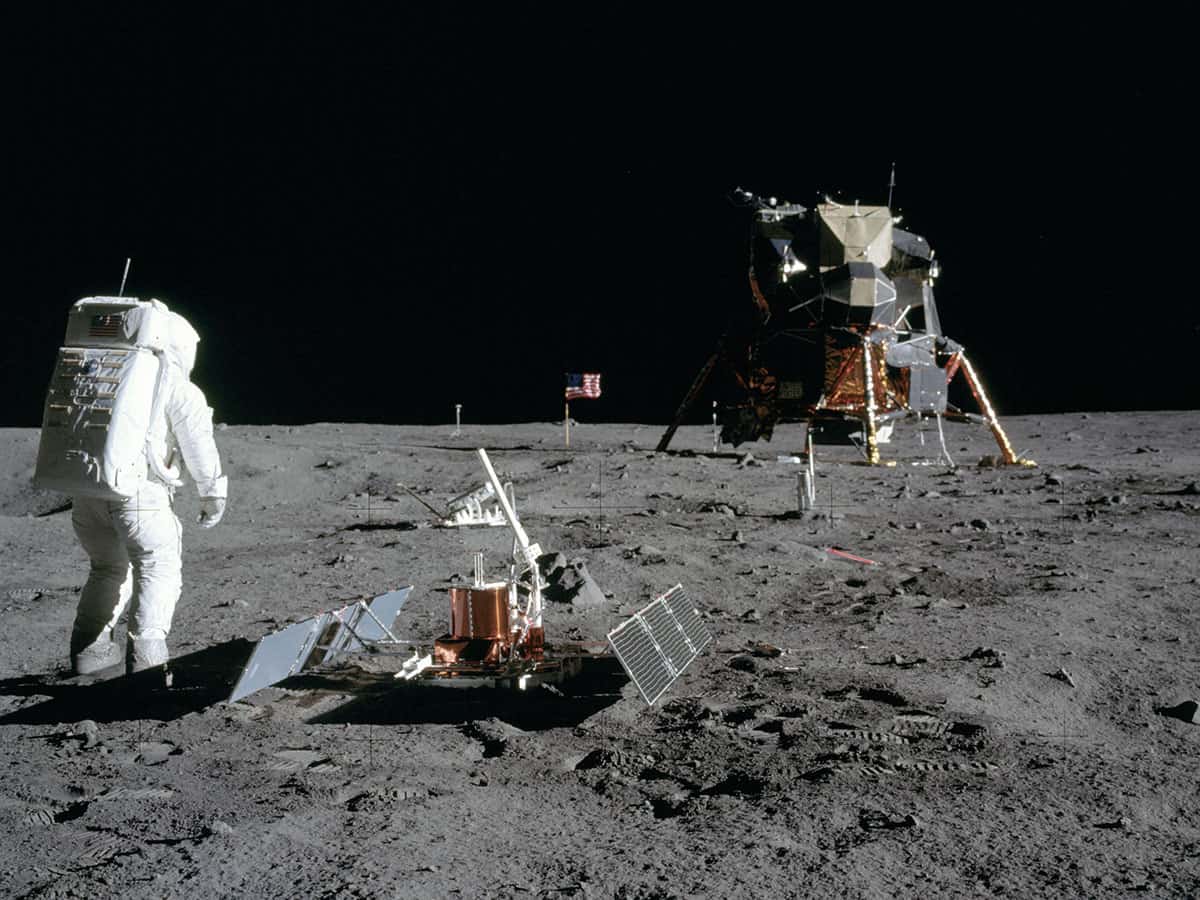
In 1965 a group of Dicke’s students approached NASA with the idea for a lunar laser ranging (LLR) effort. Endorsed in principle two years later, the students then set up the Lunar Laser Ranging Experiment (LURE) team to carry out the project. LURE initially consisted of 10 experimental physicists, but the team quickly had to bring in experts on the orbits of the Moon and planets. “We realized we couldn’t just pick that up ourselves,” says James Faller, now 85, who had been one of Dicke’s graduate students at Princeton and was then at Wesleyan University. “It’s an art form that you don’t evolve in three months.”
We realized we couldn’t just pick [LURE] up ourselves. It’s an art form that you don’t evolve in three months.
James Faller
However, the Apollo spacecraft had room for only a small amount of science. Featuring a seismometer, a magnetometer, a suprathermal ion detector, a cold cathode gauge and a solar-wind spectrometer, the craft’s Apollo Lunar Scientific Experiments Package (ALSEP) had long been fully booked. It seemed as if LURE was not going to make the cut for that crucial first Moon landing. But then, in the autumn of 1968 and with little more than six months to go before the Apollo 11 launch date, NASA engineers decided to scale back how much cargo it could carry.
In seeking to minimize the time the astronauts would have to work on the lunar surface – for nobody knew how safe that would be – Apollo 11’s experimental package had to be entirely redesigned and simplified. Renamed the Early Apollo Scientific Experiments Package (EASEP), it now included less complex versions of the seismic, solar-wind and dust-detector instruments. Crucially, however, there was now room for LURE, which had the attraction of being quick and easy to install.
All the astronauts had to do was to carry the LLR device out, set it down on the lunar surface and orient it. The instrument was simple, reliable and low-maintenance. It had no moving parts and promised numerous scientific returns. The only concern was whether Eagle would stir up dust or kick off pieces of the module as it lifted up from the Moon’s surface on its journey back to the orbiting Columbia module. If dust or debris landed on the LLR device, the experiment would be ruined.
It was a risk the engineers decided to take.
Pressure mounts
And so when the Eagle touched down on the Moon’s surface on 20 July 1969 – about 6 km from the expected landing point as the astronauts sought a smooth area to land – its cargo included a honeycomb array of 100 silica “corner cubes” measuring 46 × 46 cm in size. Each corner cube is an optical prism with three perpendicular reflecting faces – rather like three square mirrors joined at a single vertex. Together, they make incoming beams of light exit in exactly the opposite direction from which they entered (see box below).
On 21 July 1969 Armstrong and Aldrin placed this device, also known as a retroreflector, on the surface of the Moon, not far from the lander. Over the following years, four other arrays were installed too. Two slightly larger devices were placed by subsequent Apollo missions (14 and 15) in 1971, while two French-designed arrays were installed by Soviet lunar rovers in 1970 and 1973. However, the opportunity to place LURE aboard Apollo 11 had arisen so suddenly that no terrestrial telescopes were prepared to make observations.

A ranging telescope would have to be able to direct a laser beam at the retroreflector’s location and then detect the photons on their return, which was not easy. What’s more, most existing telescopes were fully booked up with other observations. But NASA urgently wanted to be sure the ranging idea could be tested while the astronauts were still on the Moon’s surface so it would know the experiment worked, Suddenly members of the LURE team were under enormous pressure to find a telescope that could do so.
The Lick Observatory in California was one option, but it was available only for about two weeks because of other astronomical commitments. Keen to have a backup in case the weather at the Lick turned cloudy, and with only four months remaining before launch, the LURE team approached Harlan Smith, director of the McDonald Observatory in Texas. McDonald had a new, NASA-funded 2.7 m telescope for observing the planets, but Smith agreed to a crash programme led by Doug Currie – another of Dicke’s former PhD students – to design and implement technology for a long-term LLR effort.
When the Eagle landed and the retroreflector was installed, the Lick Observatory was ready but McDonald was not. At the Lick, Faller tried to observe returning light pulses but with no success. One major problem was that no-one knew the exact co-ordinates of the Eagle on the Moon. “God didn’t put an X where the lander was,” says Faller. By the time the Eagle had taken off, astronomers at the Lick still hadn’t detected any signal. Then, on 1 August 1969, and with the allotted time on the observatory running out, Dicke dropped in for a visit, grilling Faller about the equipment.
God didn’t put an X where the lander was.
James Faller
That night, 11 days after the Apollo 11 landing, they saw a signal – the first bounce of a laser pulse off the lunar surface. Dicke would later refer to it as a high point of his career. On 19 August 1969 the McDonald LLR facility – operated by Carroll Alley, another former Dicke student – made its first observation, and that observatory became the LURE project’s chief data-taker from 1970 for a decade and a half. Further measurements were made from the mid-1980s onwards by researchers at the Côte d’Azur Observatory in France.
But once LURE began to measure the Earth–Moon distance with centimetre rather than kilometre resolution, a host of new factors influencing that result came into play, all of which needed to be considered if astronomers were to properly understand their measurements. Shortly after the Apollo 11 mission, Jim Williams and colleagues at the Jet Propulsion Laboratory (JPL) in Pasadena, California, therefore started putting together a model to incorporate the principal factors affecting the lunar distance. It is a model they have been improving ever since.
Williams, who is now 78 but still works part-time at the JPL, ticked off a few of the factors that affect the Earth–Moon distance when I spoke to him recently. They include the orbits and orientations of the Earth and Moon, their rotations and wobbles, and their internal forces and structure. Then there’s the size and co-ordinates of the planets and major asteroids, plus perturbations from the Sun.
The analysis has to combine elements of physics, astronomy and geophysics.
Jim Williams, Jet Propulsion Laboratory
All told, the model has about 140 parameters, Williams explained. “But it leaves out some, and there may be others,” he admits. And all of these factors had to be incorporated into a model before the lunar distance could become a meaningful measure. The analysis has to combine elements of physics, astronomy and geophysics. “It has required me to learn new things,” Williams says.
How lunar laser ranging works
Lunar laser ranging, which measures the distance between the Earth and the Moon, is simple in principle. It involves an observatory using a powerful laser to target a series of short light pulses at an array of “corner cubes” placed on the Moon. Each cube has three perpendicular reflecting faces that make the incoming light exit in exactly the opposite direction from which it entered. Peer into a corner cube and you’ll see your eye’s iris in the centre, but – unlike in a mirror – your iris will stay dead centre even when you move your head.
Astronomers typically fire 20 bursts of light every second for between three and 10 minutes, carefully timing when each burst departs. The beam is initially as wide as the telescope’s mirror – about 3 m – but each pulse spreads out in transit due to diffraction and atmospheric turbulence. By the time it reaches the Moon’s surface about 385,000 km away, the pulse is shaped like a thin pancake about 2 km in diameter. The intensity is now so weak that only a trillionth of the photons in each pancake strike the retroreflector array.
After bouncing off the corner cubes, the photons return in exactly the reverse direction, also spreading out on the journey back to Earth. By the time they have come home, the signal is fainter still. Indeed, depending on the observatory, only one return photon is detected for every outbound shot. Still, that signal is just enough to be picked up by the originating telescope, amplified, and have its arrival time recorded. Knowing the speed of light, the distance to the Moon can be easily calculated.
On the rebound
Before LLR, the Moon’s distance from the Earth was known to within about a kilometre or so. Thanks to LLR, the resolution dropped to 20–30 cm in the mid-1970s, and to 2–3 cm by 2000. That year, in an attempt to reduce the uncertainty to about a millimetre, an ambitious project was mounted at the Apache Point Observatory in New Mexico. One of the central figures in this was the University of Washington physicist Tom Murphy, who was then just 29 and not even alive when the Apollo 11 astronauts installed the first array.
While finishing a PhD at the California Institute of Technology before getting a postdoc at the University of Washington, Murphy co-wrote a NASA proposal with two colleagues to use technologies that had not been used for LLR before, including avalanche photodiode arrays to amplify the signals. The project was called the Apache Point Observatory Lunar Laser-Ranging Operation (APOLLO), and it came online in 2005. “We thought we could improve the LLR measurement by an order of magnitude,” Murphy told me. “We were excited that we could make so many improvements in so many domains.”
It turned out to be much more challenging. For one thing, at the millimetre precision level, already-known influences had to be more carefully determined, and smaller influences that could be neglected at the centimetre resolution level now had to be included. “We ran into a rat’s nest of effects,” Murphy says. “We had to account for all the influences that affect the distance between the Earth telescope and the reflector sitting on the Moon to a new degree of sensitivity.”
We had to account for all the influences that affect the distance between the Earth telescope and the reflector sitting on the Moon to a new degree of sensitivity.
Tom Murphy, University of California, San Diego
Perturbations from the Sun can shift the lunar orbit by thousands of kilometres, while Venus and Jupiter each modify the Moon’s orbit by about a kilometre. That means Murphy’s team needed to know the locations and velocities of the planets to high precision, which is difficult. “There’s no stone tablet of the positions of all the bodies in the solar system – and each has unknown parameters, including 3D position, velocity and mass,” Murphy told me. “The uncertainty quickly adds up.”
The influence and location of all the heavenly bodies in the solar system was only one issue. At the millimetre resolution, knowing the exact co-ordinates of the terrestrial observatory and the lunar reflectors now became important too – and each of these is constantly in motion. “It’s eye-opening,” Murphy says. “You think the Earth is stable, but it all moves. The Earth’s crust is pushed by groundwater, by the atmosphere, by ocean loading – bodies of water in motion – and so forth.” Indeed, when tides pile up water on the California coast it literally moves New Mexico by a few millimetres. “Each site on the Earth and on the Moon has its own personality – its own unique displacements,” Murphy adds.
Much more information about the interior structures of both the Earth and Moon needed to be established and taken into account, which is why Murphy, who is now at the University of California, San Diego, is still working on the project almost 20 years on. But at the millimetre sensitivity level, even the mirrors on the Moon’s surface are no longer stable. Over the years, the performance of the retroreflectors has been ever so slightly reduced, evidently by dust kicked up every so often on the lunar surface. “I haven’t seen it, and I’m not 100% sure,” says Murphy, “but dust is the most economic explanation. Dust absorbs light and reduces the statistics.”
Still, the new resolution has allowed LLR to achieve many of its goals. One of the earliest concerned the equivalence principle, or the notion that inertial and gravitational masses are the same. If they were not identical, the different make-up of the Earth and the Moon would mean that the Sun could accelerate these two bodies at slightly different rates, which would affect the lunar orbit radius in a way that might be big enough to measure. No violation of the equivalence principle was seen. Nor was any evidence spotted for a variation in G, which indicated no increase or decrease in the lunar distance and period (2009 Int. J. Mod. Phys. D 18 1129). The new resolution has, however, revealed much about the Moon’s interior, including the fact that it has some sort of fluid core – precisely what, though, remains unclear.
Currie – also still going strong – is among the leaders of an international group of scientists planning a Next Generation Lunar Retroreflector. It is scheduled to be carried to the lunar surface next summer by one of the commercial carriers now competing for NASA funding, and aims to improve the range accuracy by a factor of 30 and vastly expanding the scientific returns.
Contrasting legacies
Fine-tuned fundamental physical measurements are usually thought to give information about some feature of nature – the spin of the proton, the mass of the neutrino – that is disconnected, or largely so, from measures of other natural features. This is not true of the distance between the Earth and the Moon, which LLR has sought to measure. It cannot be worked out in a closed environment and is particularly intricate. Indeed, no matter how precise the instruments become, this intricacy will never go away and will become greater with each new measurement.

This new level of intricacy has also affected how the scientists involved have to work. Measuring the Earth–Moon distance requires collaborations between astronomers, astrophysicists and geophysicists, and between modellers and measurers. More and more detailed information about the positions, motions and even interior structures of all heavenly bodies have to be incorporated into understanding the meaning of each measure, while it is impossible to get a firm grip on all influences. These collaborations, too, will only get more complex still.
Fifty years on from the first Moon landing, the Apollo programme has left two strikingly different and contrasting legacies. One is the series of famous “Earthrise” photographs, starting with Apollo 8, which show a partly illuminated Earth poised just above the lunar horizon, as tranquil and implacable as the Moon seen from Earth (see image at top for the Apollo 11 Earthrise). The other, I would argue, is the LLR experiment, which is continuing to reveal ever more wobbling and juggling in the Earth and Moon, and the interaction of these two bodies with each other and with the other bodies in the solar system.
Philosophers often distinguish between “manifest” and “latent” images. The former is the world as described in the framework of ordinary human perception, while the latter is the world as described by the framework of science. Few things capture the difference better than the relation between the Earth and the Moon as depicted by “Earthrise” and by LLR. But for physicists, LLR’s many scientific returns are one reason why, 50 years after Apollo 11, it remains the only scientific instrument of that famous mission still in operation.