Gamma-ray bursts are the most violent and energetic explosions in the universe, each emitting as much energy as 100 million billion Suns. Astronomers now think that these awesome explosions occur when stars die
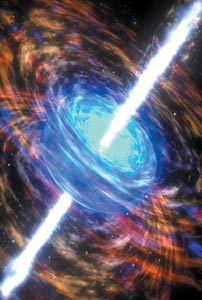
There is a Persian fairytale about the three princes of Serendip — the island in the Indian Ocean that is now known as Sri Lanka. The princes were seasoned travellers who made a series of astonishing discoveries through good fortune alone. Their luck was so amazing that we now use the word “serendipity” to describe what happens when we find something unexpectedly. As the discovery of gamma-ray bursts makes clear, serendipity often plays a key role in science.
The story began in 1963 when the world’s leading nations signed the nuclear test-ban treaty, which sought to prevent nuclear bombs from being exploded underwater or above ground. That year the US also launched the first of a series of satellites called the Velas — named after the Spanish for “watchmen” — that were designed to monitor radiation from clandestine nuclear explosions. Like the legendary princes of Serendip, the Velas made an unexpected discovery.
On 2 July 1967 one of the satellites detected a sudden bright flash of gamma rays. It then saw another from a different direction, and then another. These unexpected flashes kept coming, outshining everything else at gamma-ray frequencies for several minutes. It soon became apparent that these bursts of light were not from man-made sources and that they did not originate from the Earth, the Sun or even any of the planets.
It was not until 1973, however, that Ray Klebesadel, Ian Strong and Roy Olson of the Los Alamos National Laboratory in the US announced the discovery of what they called “gamma-ray bursts”. They waited so long to reveal their findings because they wanted to be sure that the flashes of light came from somewhere in space. Contrary to popular mythology, security considerations played no role in the delay before the findings were made public.
In the years that followed, theorists applied their minds to the mystery of these bursts, and a number of imaginative solutions were proposed. However, a lack of key observational data meant that real progress in the field only began in 1991 when NASA launched the Burst and Transient Source Experiment (BATSE) on board the Compton Gamma-Ray Observatory. Over the next 10 years it detected over 2700 gamma-ray bursts, distributed evenly across the sky (figure 1). Gamma-ray bursts clearly had nothing to do with any objects in the solar system, the Milky Way or any known nearby galaxies, otherwise they would be clustered only around certain directions.
Astronomers realized that the bursts must be caused by some sort of dramatic event — possibly an explosion. To find out more, they naturally turned to ground-based telescopes to search for possible “afterglows” of these events at optical and other wavelengths. This radiation, they reasoned, might give clues to the origin of the bursts.
Unfortunately, BATSE could not pin down the location of a gamma-ray burst to much better than 1 or 2 degrees. Such large regions of the sky — which are several times the diameter of the Moon as seen from the Earth — contain vast numbers of other objects that also emit visible light. Astronomers therefore did not know where precisely to point their telescopes to find out more about bursts that had been spotted by BATSE. Progress stalled.
Burst breakthrough
While observers were trying to detect the afterglow of gamma-ray bursts, Peter Mészáros of Penn State University in the US and Martin Rees of Cambridge University in the UK published a seminal paper in 1993 that predicted how an afterglow could be created (see further reading). They argued that any sort of explosion depositing a large amount of energy in a small amount of matter would invariably lead to a fireball travelling at very near the speed of light. This powerful jet would sweep up matter from its surroundings. Any electrons that were present would be accelerated, causing them to emit synchrotron radiation — the afterglow — at all wavelengths. Mészáros and Rees’s theory also predicted that the afterglows would fade rapidly at first and then more gradually — in other words, the radiation would follow a power law. The bursts might therefore last long enough to be observed (see Physics World April 1997 p10 and June 1997 pp23 to 24).
The breakthrough came on 28 February 1997 — almost 30 years after the first gamma-ray burst was detected — when astronomers pointed the Italian-Dutch BeppoSAX satellite in the direction of a burst that had occurred just eight hours earlier. They hoped that the on-board detectors would see a fading X-ray signal — the dying embers of the gamma-ray burst — at the very same point in the sky where the burst had been observed. Remarkably, they succeeded. This was the first direct observation of the afterglow of a gamma-ray burst and it sparked a revolution in research into these cosmic explosions.
Soon afterwards, gamma-ray-burst afterglows were found to emit not just X-rays and gamma-rays but radiation across the entire electromagnetic spectrum. However, it took another six years to establish the nature of these elusive sources. We now believe that gamma-ray bursts are the most energetic explosions that stars ever experience — even more powerful than supernovae — and that they can be seen from the Earth across the entire volume of the visible universe.
Hunting down gamma-ray bursts
If you look at the night sky with a powerful optical telescope, you will find that it is lit with millions of dots. You would have a very hard time trying to pick out gamma-ray bursts among all these other objects. Most astronomers therefore search for the bursts at gamma-ray wavelengths, where such sources are few and far between. Following the retirement of BeppoSAX in 2002, most gamma-ray bursts are currently detected by the US’s High-Energy Transient Explorer (HETE-2) and Europe’s International Gamma Ray Astrophysics Laboratory (INTEGRAL).
However, astronomers have to act quickly because gamma-ray bursts fade relatively fast. Once a burst has been roughly located to arc-minute accuracy with one of these satellites, its position is immediately distributed to astronomers and observatories around the world via the Gamma-Ray Burst Coordinates Network (GCN). The burst is then pinned down to arc-second accuracy either at X-ray wavelengths using the Chandra X-ray Observatory or the XMM-Newton satellite, or using ground-based optical or radio telescopes, such as the Very Large Array (VLA) in New Mexico.
Astronomers know that they have tracked down an afterglow when they find a previously unknown source with an intensity that decays with time (figure 2). They can then carry out detailed studies of the light from the burst using spectrographs or optical CCD cameras. The afterglow usually lasts for days to weeks at optical and X-ray frequencies — and months in the case of radio waves. Observers therefore have some time — although not much — to obtain good-quality optical spectra.
These spectra often have absorption lines, which indicates that certain wavelengths have been absorbed by matter as the radiation travels from the source of the burst to the Earth. By measuring how much these lines have been red-shifted to longer wavelengths as a result of the expansion of the universe, astronomers can determine how far away the burst is. The immediate and irrefutable result of this work is that gamma-ray bursts originate in galaxies at cosmological distances — sometimes even near the edge of the observable universe. The most distant burst ever detected was spotted on 31 January 2000 by Michael Andersen of the University of Oulu in Finland and his colleagues at the University of Copenhagen, including one of us (JH). Its light was emitted when the universe was just 1.4 billion years old — 10% of its current age.
Gamma-ray bursts are so distant and have such high fluxes of radiation that their total energy output is huge. For example, gamma-ray burst 990123, which was seen on 23 January 1999, was initially believed to have released about 4 x 1047 J of energy — more than would be generated if the entire mass of the Sun or a neutron star were converted into gamma rays. This figure seems absurdly high, because we know of no credible mechanism that could convert such a huge mass into gamma rays in a matter of seconds. However, gamma-ray bursts do not emit equal amounts of energy in all directions, as astronomers had previously assumed. If the radiation is emitted from just a very small part of the fireball (like a lighthouse beam) a quick recalculation reduces its total energy by a factor of 100-1000. The energy output is now in the realm of the believable — typically 1044 to 1045 J — which is not much more than that of a supernova.
Astronomers soon gained corroborative evidence that the outflow from a gamma-ray burst is collimated in this way. The most compelling evidence came when they found that the afterglow starts to fade more quickly with time, which hinted at changes in the geometry of the emitting regions. What seems to be happening is that the jet that produces the afterglow expands as it slams into matter that surrounds the source. It therefore slows down and beams its radiation to a larger angle. The beam becomes less collimated as it expands, causing the afterglow to start to fade more rapidly. This sudden drop, or break, in the afterglow light curve occurs at all wavelengths and can be used to calculate the angle of the initial cone of radiation and hence the total energy released at the source.
But if we are only observing emissions from a narrow cone that happens to be in our line of sight, then surely there must be hundreds of other gamma-ray bursts that we cannot see because they shine in other directions. So how often do they really occur in the universe? The jury is still out on this question, but the true number is probably hundreds — and possibly even thousands — per day in the observable universe.
The supernova connection: a one-two punch
In 1993 one of the present authors (SW) wondered if gamma-ray bursts are linked to supernovae — bright explosions that occur during the death-throes of stars that are at least eight times more massive than the Sun. When such stars deplete their nuclear fuel, they no longer have enough energy and pressure to support their mass. The core implodes, forming either a neutron star or — if there is enough mass — a black hole. The energy released by this collapse flings the surface layers of the star outwards, creating a bright explosion. Although most supernovae cannot produce gamma-ray bursts because their explosion velocities are too slow to make gamma rays, a special type of supernova — in which most of the energy is transferred to jet-like outflows along the rotation axis — could produce the fireballs that are believed to create gamma-ray bursts.
True to the call, an unusually energetic supernova was detected in late April 1998, named SN1998bw. It appeared to be near a gamma-ray burst that had occurred just a few days earlier on 25 April. Astronomers argued that the probability of this happening by chance was less than 0.01%. SN1998bw appeared to provide tantalizing evidence for a possible link between supernovae and gamma-ray bursts. But could the researchers really be sure? Many astronomers were sceptical. This particular gamma-ray burst, they argued, was an odd one, being several orders of magnitude less energetic than common gamma-ray bursts. The supernova itself was also unusual, they said.
It was not until last year that we could confidently say that we had found the “smoking gun”. On 29 March 2003 the HETE-2 satellite observed, in the constellation Leo, one of the brightest and closest gamma-ray bursts on record. Ground-based follow-up observations of its afterglow revealed, for the first time, that a gamma-ray burst and a supernova had occurred essentially simultaneously — a quick and powerful one-two punch. GRB 030329 occurred only two billion light-years away (Physics World May 2003 p3). The burst lasted about 30 s and it was among the top 10 brightest bursts ever recorded to date. Its afterglow lingered for weeks at lower-energy X-ray, radio and visible wavelengths.
However, only direct observational evidence would prove once and for all that gamma-ray bursts and supernovae are linked. Working with members of the international Gamma-ray Burst Afterglow Collaboration at ESO (GRACE), the present authors decided to monitor the afterglow to see if we could detect the characteristic spectral features of a supernova in the fading light of the burst. (A competing group
led by Peter Garnavich of the University of Notre Dame and Thomas Matheson and Kris Stanek from the Harvard-Smithsonian Center for Astrophysics also carried out the same test.) Within days, we had discovered broad lines in the optical afterglow that were very similar to those seen in the spectrum of supernova SN1998bw. These lines indicated that we were witnessing a massive, rapidly expanding supernova shell, sometimes called a “hypernova”, that was moving as fast as 12% of the speed of light. Projecting these expansion velocities back in time, we concluded that the supernova of 29 March 2003 had indeed exploded at the same time as GRB 030329. The link between supernovae and gamma-ray bursts was proven.
The gamma-ray burst that took place on 29 March 2003 changed everything for researchers in the field. Astronomers are now sure that at least some gamma-ray bursts are produced when black holes — or perhaps very unusual neutron stars — are born inside massive stars. Gamma-ray bursts are so bright that, during the fleeting moments of their existence, they emit as much energy as 10 million billion Suns.
But what exactly happens during this process? Although Mészáros and Rees’s fireball model is still the most popular explanation of the essential physics of the afterglow, it and other competing models deal with the aftermath of these explosions. The best contender for how such a fireball is created in the first place is known as the “collapsar” model, which was first developed by one of us (SW) back in 1993 and explored further with Andrew MacFadyen, who is now at the California Institute of Technology (figure 3).
How gamma-ray bursts are made
The story starts hundreds of thousands of years before the explosion that creates the gamma-ray burst when a massive gaseous star runs out of fuel and sheds its outer envelope of hydrogen and helium. Driven off by the star’s own radiation, or perhaps lost to a binary companion star, the loss of the envelope turns the star into a bluish compact star. The remaining star — containing about 15 solar masses worth of helium, oxygen and heavier elements — quickly loses its remaining fuel and its inner core collapses to form a black hole. At the same time, parts of the star pile up in orbit around the black hole to form an accretion disk, while the outer layers of the star respond more slowly, unaware of the catastrophe in progress at its heart. Powerful electric and magnetic fields are generated because the inner part of the disk rotates much faster than the edge, perhaps amplified by the rapid rotation of the black hole itself.
These fields launch jets of matter that flow out along the rotational axis of the star. They burrow with ease through the outer parts of the star, taking about 10 s to get there. Meanwhile, winds of super-hot neutrons and protons blow away from the disk. As the winds cool, these particles reassemble to form tightly bound nuclei such as nickel-56. The winds and the jets shatter the star in a gigantic explosion — a supernova. About an hour later, far outside the original star, collisions occur between pieces of jet that are moving at different velocities near the speed of light . These collisions are responsible for the gamma-ray burst, flinging radiation in our direction. Days and weeks later, the nickel-56 decays into cobalt-56 and iron-56, producing the energy that makes the supernova shine so bright.
X-ray flashes
If the outflow from a collapsar is indeed collimated, then perhaps just one in every few hundred of the ensuing jets will be aimed at the Earth. Could it be that there are many more explosions that we have not yet seen, caused by jets that are not pointing at us? If so, what would they look like?
The collapsar model provides the answer. We now know that the matter in the jets of a gamma-ray burst is ultra-relativistic — in other words, it is moving at about 99.999% of the speed of light. Depending on the angular structure of the jet, off-axis emissions are produced by somewhat more slowly moving matter. This material will be less affected by the Doppler effect and its intensity will therefore peak at a lower frequency (and hence lower energy) than the on-axis emissions.
So if we want to spot gamma-ray bursts caused by off-axis emissions, we should look at X-ray frequencies. Remarkably, nature complied. Shortly before the BeppoSAX satellite retired, it discovered brief flashes of X-rays that had very little accompanying energy in the gamma-ray regime. These flashes have since been confirmed by the HETE-2 satellite. Could these X-ray flashes be gamma-ray bursts seen slightly off-axis?
To find the answer, we need to consider what fingerprints these flashes would leave on our data. First, we can expect the afterglow to be invisible initially, since it points away from us. It will then come into sight as the outflow slows down, before decaying like the afterglow from any other burst. Second, if X-ray flashes and gamma-ray bursts are two manifestations of the same physical phenomenon, and if gamma-ray bursts are associated with supernovae, then X-ray flashes should also be accompanied by supernovae. The third clue comes from the fact that off-axis emissions are less intense than head-on gamma-ray emissions: we will therefore tend to spot only those X-ray flashes that are particularly bright — and hence closer — than conventional gamma-ray bursts. Finally, we can expect to detect more X-ray flashes than gamma-ray bursts.
All of these properties were exhibited by an X-ray flash that was observed by detectors on-board the HETE-2 satellite on 23 July 2003. Its optical afterglow was found by Derek Fox and colleagues at Caltech using the Palomar 60 inch telescope. Its light curve was measured by Johan Fynbo and colleagues at the Niels Bohr Institute in Copenhagen, working with members of the GRACE collaboration (figure 4). More and more phenomena are now being tied together in the overall picture of gamma-ray bursts and it is becoming clear that the bursts are a motley crew of explosions transcending the gamma-ray astronomy field.
Swift progress expected
Although there has been great progress in our understanding of gamma-ray bursts over the last few years, the mystery has still not yet been fully solved. New light could be shed on the puzzle by a NASA-led international satellite named Swift, which will be launched this month (see news story on page 12 print edition only). It will be able to locate gamma-ray bursts within seconds of them being observed. Once an event triggers an on-board telescope, the satellite will automatically and “swiftly” repoint its own X-ray, optical and ultraviolet instruments at it. With the mission likely to pinpoint as many as 100 bursts every year, afterglow hunters will be busier than ever.
One of Swift’s aims is to study a type of gamma-ray burst that was first observed by one of us (CK) and her colleagues at NASA’s Marshall Space Flight Centre in Huntsville, Alabama, using BATSE in 1993. This team found that some gamma-ray bursts last for no more than 2 s and contain relatively large number of photons at high energies. These “short” bursts are very different from the more familiar “long” bursts. About a quarter of gamma-ray bursts are of the short variety, but none of them has been accurately located, leaving the question of where they come from unanswered. We do not know if they are associated with the deaths of massive stars — or even with galaxies. Are they a variant of collapsars or perhaps merging neutron stars? A crucial test will be to look for afterglows as well as possible supernova signatures in their afterglows.
Thanks to its highly sensitive instruments and large field of view, Swift will also be able to detect many more faint gamma-ray bursts — both on- and off-axis — than previous satellites. Some of these bursts will be intrinsically faint, but some will be dim because they are simply very far away. These bursts will therefore be extremely important tracers of star formation, enabling astronomers to probe the physical conditions of the universe when the first stars were forming (see “Star formation” on pages 25 to 29 print edition only). Gamma-ray bursts could therefore tell us not only about how massive stars died, but also how they were born.
As an added bonus for those who get their thrills from stellar explosions, Swift will also be a splendid supernova hunting machine, given that we now know that the two phenomena are inextricably linked. In particular, it will be able to detect a significant population of intrinsically faint bursts at modest distances. As these are more likely to be off-axis than on-axis, the supernova will not be hidden by the bright afterglow of the burst. It should therefore be easy to study how supernovae evolve in the first few minutes, days and months after the burst. Swift will also let us study supernova explosions very soon after they occur and detect many more Type Ic supernovae, providing an unprecedented insight into the geometry of supernova explosions.
Swift promises to herald the start of a fascinating new era of research.