Optical tricks that have given butterflies, beetles and other creatures an evolutionary advantage are beginning to teach physicists a thing or two about advanced photonics, as Pete Vukusic explains

The ability to manipulate and control light is one of the foremost goals of modern optics. However, it is also something that nature perfected a long time ago. About 500 million years ago an evolutionary “big bang” took place, when animal life began to rapidly diversify. This so-called Cambrian explosion is thought to have been triggered when the manipulation of light began to influence the survival of many animals. When it comes to highly advanced optical systems, it seems there is no more experienced an engineer than nature itself.
It is little wonder, then, that the optical engineers of today have started to keep a curious eye on natural optical systems. These systems offer inspiration for new ideas in optical design and could provide shortcuts for developing advanced photonic systems, which one day may even be grown to order. Without doubt, uncovering nature’s optical secrets has been, and will continue to be, a spectacular journey.
Early beginnings
Some of the most interesting optical systems in nature are hard to miss. They are often brightly coloured, metallic looking or strongly iridescent, and stand out from ordinary objects and surfaces that are coloured by pigmentation alone. In the 18th century Robert Hooke and Isaac Newton were among the first to explain the underlying physics of these systems. They correctly predicted that the iridescent colours of peacock feathers and silverfish scales resulted from their physical structure rather than pigmentation. This was a huge leap of understanding, considering that the means of seeing these structures would not be invented for a further two centuries.
It was left to James Clerk Maxwell in the 19th century to develop the set of mathematical tools that now enable us to fully describe the way electromagnetic radiation interacts with matter. This interaction happens all the time, of course, but it starts to get interesting when it takes place in the presence of structural periodicity. Under the right conditions, periodic structure modifies the character of the light that is transmitted, reflected or absorbed by a material. Roughly speaking, the size of the periodicity has to be about the same size as the wavelength of the light involved. An object with a periodicity of a few millimetres to a few centimetres, for example, could control the propagation of microwaves, while the control of optical wavelengths requires periodicities of tens to hundreds of nanometres.
One very important optical constraint for a material, besides its refractive index, is the number of dimensions exhibited by its periodicity. This offers the potential for light to be controlled in one, two or three directions. If 1D or 2D periodicity is limited to the surface of an object, for example, the structure is commonly known as a diffraction grating. If 2D or 3D periodicity exists within the bulk of an object, however, it becomes what is now called a photonic crystal. It is so named because light interacts with the structure in a manner that is analogous to the way that electrons interact with a periodic crystal of ions. Both these processes result in specific bands of allowed energies that are separated by band gaps – regions in which no states can exist. For photonic systems, these are called photonic band gaps and they underpin the way the flow of light is controlled.
Over the last 10 years or so the physics of 2D and 3D photonic crystals has been examined in great detail, and there is currently significant interest in optical technologies based on photonic structures. Synthetic photonic-crystal fibres – which were pioneered by Philip Russell and co-workers at the University of Bath in the early 1990s – could outperform even optical fibres in their ability to transport light (see “Photonic band gaps deliver the goods”, October 2003).
Nanostructure in nature
Nature learned relatively early about the way light interacts with periodic structures due to the evolutionary selection advantages it offers. Highly reflective and coloured surfaces can play an important role in courtship, or in signalling warnings to predators. The first such optical systems appeared as surface diffraction gratings that have been found in Burgess Shale fossils that are 500 million years old. These comprise near-periodic and parallel corrugations on the external hard surfaces of the creatures. Similar periodic systems are also found in some common present-day insects, such as wasps, flies and moths.
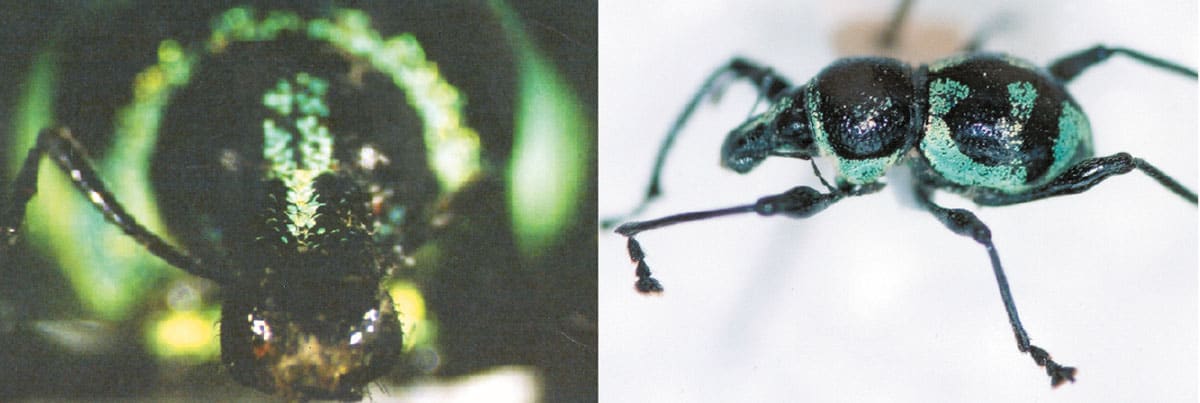
Natural surfaces with 2D gratings are not normally designed for bright-coloured reflection. Indeed, provided they comprise the right sort of nanostructure, such gratings can actually reduce the reflection of light at interfaces. Instead, they are found to form antireflection surfaces on many insect eyes, which increase the efficiency of the animal’s vision and reduce unwanted reflection that might attract predators.
Periodicity within materials, rather than on their surfaces, underpins the vast majority of all structural colour in nature (figure 1). This is the case for the 1D multilayer systems responsible for the characteristic colours we associate with nature’s brightest reflectors: butterflies, birds and fish. The materials that make up these multilayers depend on many things, not least the inherent physiology, ecology and environment with which the animal is associated.
1 Periodic design
Natural systems contain nanostructure in a broad variety of structural designs, which can exhibit periodicity in one, two or three dimensions. (a) 1D periodicity in the form of multilayers can be more-or-less flat and continuous, which is the structure responsible for the iridescent colouring in some beetles, plants and diurnal moths. (b) It can also be discretely packaged, such as that found in Morpho butterflies and certain iridescent plant leaves. (c) Some 1D structures contain fibres the orientation of which are continually rotated through the layering. (d) When three individual filters that reflect different colours are stacked on top of one another, they can collectively have a silver or gold appearance. Layers with various thicknesses arranged in a pseudo-chaotic fashion (e), and those arranged in a chirped manner (f), also produce the effect of broadband reflection. These three broadband reflector designs are found in various silver- and gold-coloured fish and insects. (g) Natural 2D periodicity generally comprises close-packed rods of solid material surrounded by air or a liquid with a lower refractive index, which can be found in cat’s eyes and some iridescent bird feathers. (h) 2D periodicity also comes in the form of cylindrical voids that are embedded in a high-refractive-index solid medium, such as those found in the iridescent hairs of certain marine worms. Two principle variations of 3D periodicity are known to exist naturally. (i) Close-packed spheres of solid material generate the iridescence of gem opals and have recently been discovered in certain beetles, while lattices that are filled with hollow voids generate the iridescence of several species of exotic butterfly (j).
In some butterflies, for example, layers of cuticle are intercalated with layers of air in order to generate efficient interference colours, while in many aquatic vertebrates such as fish, the same trick is performed by platelets of solid guanine separated by cytoplasmic fluid. The occurrence of 1D multilayers is extremely common in insects, and a great many of these systems have been investigated thoroughly over the last 40 years. Furthermore, these structures are not limited to fauna but are also found in some plant leaves, berries and algae.
It is difficult to say how long ago selection pressures led to the evolution of 2D and 3D periodic structures within systems. Compared with 1D multilayers they are rather uncommon, and 2D bulk periodicity is most often found in the form of long fibres. Recently Andrew Parker of Oxford University and David McKenzie of the University of Sydney decided to characterize the structures of the hairs of the Aphrodite – a type of worm that inhabits shallow tropical water. What they found was a 2D photonic-crystal fibre.
Each Aphrodite hair contains thousands of hollow, close-packed and longitudinally oriented cylinders of cuticle, each with a diameter of about 230 nm. Bright colours are produced when the cylinders collectively diffract light that is incident on the side of the fibre. Theoretical models suggest that if the structure contained a centrally located defect – such as that in synthetic photonic-crystal fibres – it would be able to guide light down its length. The absence of such a defect, however, suggests that the purpose of these structures is instead for iridescent signalling.
Full 3D periodicity was first investigated in detail in the 1960s, when it was realized that such a structure underpins the iridescence of gem opals. John Sanders, formerly of the Commonwealth Scientific and Industrial Research Organization in Australia, characterized the close-packed arrangement of silica spheres that make up opal structures, and which interact with light to produce their characteristic iridescent colour. More recently, Andrew Parker and co-workers have discovered an analogous 3D sphere structure in a beetle.
A variation of the opal structure – which is sometimes referred to as inverse opal – has been known to exist in certain butterflies for some time, and has been studied in detail by the author and colleagues in the photonics group at Exeter University in the UK. Instead of close-packed spheres, the butterfly structure comprises a lattice of hollow air-filled voids that are formed within a network of interconnecting cuticle. Given the current technological interest in synthetic 3D photonic crystals, these natural 3D systems are quite astonishing for the very fact that they have developed the way they have. The structure appears to be a minor variation of the diamond-like tetrahedral structure, which offers excellent reflectivity over a broad angle range for the particular difference in refractive index between air and the butterfly cuticle.
To further enhance the angle-independent colour, the 3D structure usually appears divided into domains that are about 5 μm in diameter. Each neighbouring domain has the same structure, but they all face in slightly different directions with respect to each other. As a result, the average overall colour effect is essentially angle-independent. Whereas the production of gem opals is understood to be an artefact – a by-product of the way precipitative silica-gel solution settles – the 3D photonic crystals in butterflies and beetles have evolved for a specific biological purpose, which is thought to be associated with visibility.
Natural periodic structures tend to be associated with high reflection or transmission, but creatures have been discovered that can control light in other ways too. Joanna Aizenberg and co-workers at Bell Labs in the US have characterized a 2D surface array of microlenses on the arms of a light-sensitive brittle star – an animal that resembles a starfish. Each lens is a photonic element that is formed from a single anisotropic calcite crystal, and has a characteristic double-lens design with a focal length of a few microns.
The size and profile of each lens is ideal for the task of collecting and focusing light. Their surfaces are shaped in such a way that spherical aberration is minimized, which means that each lens focuses light to a sharply defined point. Furthermore, the orientation of the constituent calcite crystal removes the effect of birefringence, which would otherwise cause different polarizations to behave in different ways and therefore diminish optical function. By focusing incident light onto photoreceptors beneath them, this astonishingly elegant microlens array provides the means to sense light discretely across the arm of the brittle star.
In the completely separate kingdom of plants, analogous but less specialized microlens arrays have evolved in some shade-adapted plants to increase the efficiency of photosynthesis.
Optical nanotechnology and biomimetics
Biomimetics is the extraction of useful design principles from systems that work well in nature. However, humankind’s development of optical systems over the last 100 years reveals something very curious: of all the optical systems developed so far, virtually none appear to have been the direct result of optical biomimetics. We seem to have expended incredible time, effort and expense to produce systems that nature has been using for millions of years.
One of the best examples of this is the traditional multilayer filter, in which layers with alternate high and low refractive indices control the reflection and transmission of light by means of interference. Multilayer filters are an intrinsic component of lasers, telescopes, gravitational-wave detectors and medical probes, but understanding them has developed completely independently of any knowledge of the multilayers commonly found in butterflies and birds.
It is only recently that interdisciplinary research has brought about dramatic discoveries associated with natural optical systems. Despite detailed work from the 1960s onwards by eminent biologists and zoologists, few physicists paid much attention. The analysis of the photonic-crystal-fibre structure of Aphrodite hairs, for example, was taken seriously by physicists because it had strong similarities with the synthetic photonic-crystal fibres developed by physicists a few years earlier.
However, when a little-known scientist named Wolfgang Schmidt looked at the iridescence associated with the hairs of the Aphrodite in the 1940s, the state of optics and related technologies was such that its importance, and indeed elegance, was entirely overlooked. Similarly, recent studies of the 3D photonic-crystal structure of certain butterflies and moths by the author and co-workers at Exeter were preceded by the work of several biologists in the 1960s. Back then, the implications of a natural 3D structure that could effectively control the flow of light in all three dimensions was simply not appreciated.
Today, with tens of thousands of scientists striving to develop optical technologies for a broad variety of applications, we are much more aware of nature’s optical achievements. Taking this one step further and actually learning from nature is, however, only just beginning, and the science of optical biomimetics is very much in its infancy.
Biomimetic myths
Due to popular myth or otherwise, the origins of some modern optical technologies are widely attributed to inspiration from natural optics. In only very few exceptions, however, has this been the case. Take the current use of antireflective materials to enhance the transparency of certain surfaces, in which a smooth and gradual change in effective refractive index is introduced across the interface between two media. Since most transparent glass or plastic is actually not completely transparent – reflecting up to about 8% of light at normal incidence and even more at grazing incidence – improving its transparency can increase the efficiency of, say, a solar cell, that is housed beneath it. This principle, in fact, already enhances the solar gains of some commercial architectural glazing and solar collectors (figure 2).
2 Insect eyes
Insects have already perfected the art of designing antireflective surfaces. (a) Antireflection nanostructures on the lower section of a glass sheet remove the glare that is observed near the upper edge. (b) The antireflective surface region is coated with a nanostructured porous Sol-Gel film, which was produced using the ORMOCER deposition technology and comprising hybrid inorganic and organic composite materials. (c) These synthetic structures are very similar to the natural antireflection nanostructures that are found on many insect eyes, and on transparent wing surfaces. (Courtesy: Fraunhofer Institute for Solar Energy Systems)
As a technology, however, antireflection borrowed little from nature’s expertise. Knowledge of the antireflective effect of the graded index offered by such discrete nanostructure arrays was well known even in the 19th century – long before the development of the electron microscopes required to image them. Even after the advent of the electron microscope, direct biomimicry was not pursued because antireflective periodic structures had already been developed for microwave frequencies. It is no surprise that these had a similar ratio of wavelength to pitch as the antireflective optical nanostructures found in insect eyes.
Where else, then, are the similarities between synthetic and natural optical systems so close that it appears optical biomimicry has occurred? Perhaps an unusual suspect lies in textiles. Some researchers are attempting to link visual and tactile preferences in cloth fibres with fluctuations in the periodicity of their surface irregularities. This has recently led to a new class of textiles – which are referred to in Japan as shin-gosen products – that are more desirable due to their attractive textures and their visual properties.
One particularly intriguing aspect of this research involves fibres of “odd cross-section”. Specialized techniques of extrusion-based manufacturing modify the cross-section of the fibre, which not only allows the fibres to be close-packed but also to produce optical interference and scattering from fine periodic structures within each fibre structure. Curiously, these fine structures also improve the material’s wettability, since capillary forces in the inter-fibre volume are sufficient to carry away liquid perspiration. A designated periodicity within each fibre cross-section, akin to the periodicity associated with the nanostructure on an iridescent Morpho butterfly scale, creates strong colour effects using interference.
A separate range of cloth fibre effects are evident in a product called Microcratered Fibres. The surface of these cloths is uneven on a scale of a few hundred nanometres, which minimizes the scatter of stray light. This property is able to emulate the depth of shade that is associated with quality wool-fibre yarns using only polyester materials. Although its surface very closely resembles that of many black insect surfaces, it is a design that has once again been developed independently of any inspiration from nature. Interestingly, the generic micro-crater design also forms the basis of the ultra-black, metal-alloy surfaces recently developed by Richard Brown and colleagues at the National Physical Laboratory in the UK.
Natural security
But there is an area in which optical biomimicry is starting to work: anticounterfeiting. Many of the recent security measures that have been introduced on banknotes, credit and debit cards, and branded goods rely on complex optical logos, insignia and colour schemes. Nanostructure is pivotal in nearly all these measures (see figure 3), some of which even comprise specialized pigments such as the optically variable ink (OVI) developed by SICPA of Switzerland.
3 Light security
Nanostructure-based anticounterfeiting measures exist to protect various forms of currency, documents, and bank and identity cards. (a) The optically variable devices of OVD Kinegram Technology in Switzerland are among the most effective measures, shown here incorporated in an ID card. Another successful technique (not shown) is to use specialized inks that are deposited in layers, which create iridescent angle-dependent colour effects that can be used to greatly reduce the risk of illegal copying (see text). Natural systems offer ideas for future anticounterfeiting measures. The blue and yellow juxtaposed regions shown in (b), for example, create the overall green appearance of the bright green butterfly wing in (c) through a highly specialized multilayer design system. (d) The multilayer cross-section is responsible for one of the small regions in (b) that comprises a blue annulus surrounding a yellow centre. In addition to the colour effect shown here, the multilayer produces a strong polarization effect that cannot be seen in the image. (Courtesy: OVD Kinegram AG, Switzerland; Nature)
The OVI technique exploits advanced screen-printing methods to produce ink layers of materials with alternating refractive indices, which results in specific iridescent patterns. Other “pearlescent” inks made from clear varnishes are also used. Here, the varnishes are infused with mica flakes that are coated with interference layers of iron and chromium oxides with high and low refractive indices, respectively.
Diffractive, optically variable devices – such as foil patches, stripes and windowed thread – have led to reasonably effective security features on banknotes throughout the world, but they tend not to match the durability of the banknote substrate itself. Embossed holograms and kinegrams have also been used to authenticate bank and credit cards for many years. However, the security they provide is limited by their low surface relief (typically 0.25 μm). This makes the authentication susceptible to counterfeiting because the complete holographic microstructure can be exposed by stripping the hologram from the substrate. Counterfeit tooling can then be made with only the minimum of expertise.
A big improvement in counterfeit deterrence can be gained by the use of high-precision, non-holographic micro-optics and nanostructures, which not only have a surface relief of a few microns but also have sub-surface structure. Some natural optical systems fall straight into this category of micro-optic structure. Chris Lawrence and colleagues at QinetiQ in the UK have been taking specific nanostructure designs directly from butterflies and moths, and trying to replicate them for use as security features in currency.
One specific structure, which was originally characterized by our group at Exeter, comprises a single sculpted multilayer that produces microscopic arrays of two individual structural colours. These colours are juxtaposed in such a way as to produce the appearance of a third colour, in the same way that a colour television creates the full colour spectrum by adding together three individual colours with different intensities. But the specific design of the multilayer of this particular butterfly assigns anomalous polarization properties to only one of the two individual colours. In other words, the reflection of one colour is polarized, while that of the other colour is not. This offers a covert and additional security feature that considerably decreases the risk of counterfeiting.
Designer photonics
Of particular interest in the long term is the way that natural nanostructured systems are self-assembled. In animate systems these schemes are put together in the egg, pupa or as part of normal growth in juveniles. Their formation is therefore controlled at the genetic level, but it also appears to be influenced by external factors such as temperature and nutrition. Other factors, such as exposure to X-rays and laser-mediated heat shock, are also known to alter the development of pigment and structure in specific ways.
Many groups, such as those of Paul Brakefield at Leiden University in the Netherlands and Antonia Monteiro of Buffalo University in the US, are now investigating how variation at the DNA level in developmental genes determines external optical appearance. Once this is completely understood at a fundamental level, it might be possible to change the genetic make-up such that designer nanostructures could be cultured. This is certainly a long way off, but the possibilities are fascinating.
Imagine cultivating a made-to-order 3D photonic crystal on or within an organic light-emitting diode to improve its efficiency, or as part of an optical micro-electromechanical device to improve functionality. Or imagine covering the glass surface of a solar cell with a gene-rich solution that would grow into an antireflective nanostructure array, or onto the surface of a hybrid lens in an advanced camera for it to grow into a large-area microlens array. More useful still, consider the advantages of being able to grow natural photonic-crystal fibres that can guide light, or other 2D crystal structures for optically integrated circuits in all-optical computers.
Rich rewards lie in a variety of established and emerging technologies, not only in taking the design rules and paradigms offered to us by nature’s optical systems, but also in trying to take advantage of some of its self-assembly processes. We have just begun to appreciate how advanced natural optics can be. Even some of our most recent developments in photonic systems, such as photonic crystals and fibres, have in principle already existed for a very long time.
Physicists and engineers would do well not to shy from biological systems but to embrace some of the ideas they offer. There are undoubtedly more lessons to be learned in nature’s optical arena than have come to light in the last few decades. After 500 million years of optical R&D, this is bound to be the case. We should not be surprised at what we find. Given enough time, evolution can do some pretty amazing things.
- This article first appeared in the February 2004 issue of Physics World.