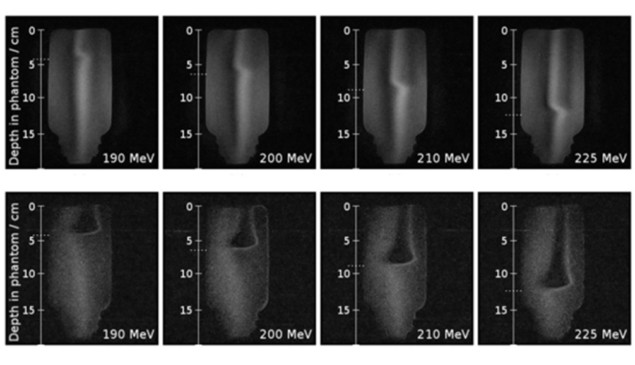
Proton therapy holds the promise of delivering highly conformal dose distributions to a target tumour volume, due to the finite range of the proton beam. However, the accuracy of this delivery can be affected by factors such as target motion, which may lead to overdosing regions of healthy tissue and underdosage of the tumour volume.
“Proton therapy is very sensitive to changes in the position and shape of the patient as a whole and the patient’s organs, both between fractions and within one fraction,” explained Sonja Schellhammer, recipient of the Donal Hollywood Award for the best abstract presented at the recent ESTRO 2020 congress. “It would be ideal if we could monitor the proton range simultaneously with the patient anatomy in real time.”
Currently, verification of proton range is mostly based on detection of secondary radiation such as prompt gamma rays or acoustic waves. Another indirect approach involves using MRI to visualize long-term radiation-induced biological effects. MRI benefits from excellent soft-tissue contrast, offers real-time imaging, delivers no radiation dose and could prove ideal for integration with proton therapy.
With this in mind, scientists at OncoRay/Helmholtz-Zentrum Dresden-Rossendorf (HZDR) are working to integrate proton therapy with MRI. Previous studies by others have shown that MRI can determine proton beam range using an off-line approach, with contrast-enhanced images recorded weeks or months after irradiation. “The question we asked in this study, is whether it would be possible to visualize the proton beam with online MRI during irradiation,” Schellhammer explained in her ESTRO presentation.
To test this idea, the researchers designed a phantom experiment using the Dresden proton therapy facility, where OncoRay, in collaboration with IBA and ASG Superconductors – Paramed MRI Unit, had integrated an open 0.22T MRI scanner with a fixed proton research beamline. The proton beam was incident upon a plastic bottle filled with deionized water and placed in the scanner’s magnetic isocentre. The team used a PMMA range shifter to tailor the proton energy such that the beam stopped within the bottle, and recorded MR image slices through the centre of the beam.
The researchers performed one scan during proton irradiation, and three immediately after irradiation, using a variety of MR sequences. For two of the six MR sequences – the proton density-weighted gradient echo (GE) sequence and the inversion recovery gradient echo (IRGE) sequence – they saw signatures in the MR image that were likely induced by the proton beam. They also observed that these signals didn’t disappear immediately after irradiation, but faded over tens of seconds.
“With this experiment, we confirmed that there is a measurable proton beam-induced signal on the MR images,” said Schellhammer. “We then asked whether this could be useful for range verification.”
Next, the team irradiated the water phantom using proton beams with energies of between 190 and 225 MeV (corresponding to different beam ranges). The observed MR signal changed in depth with increasing range, and the measured residual ranges agreed with the calculated values to within 2 mm.
Repeating the experiment with four different beam currents (1, 3, 9 and 27 nA, corresponding to dose rates of 1.7, 5, 15 and 45 Gy/s) demonstrated that the signal intensity increased with increasing beam current. MR images (recorded for 20 s) were only visible using beam currents of 3 nA or more.
To investigate whether this approach may work in a patient, the team used the same experimental set-up to irradiate other liquid phantoms (ethanol and petroleum), highly viscose materials (sugar syrup, mayonnaise and gelatine) and a tissue-mimicking material (a pork chop). While images of ethanol and petroleum showed similar beam-induced signals as seen with water, for the more viscose materials and the pork chop, no signal was seen.

MR-guided proton therapy: a status update
“It appears that the signal is only present in liquids, so likely may not be transferable to patients,” explained Schellhammer. “But this approach may well prove useful for quality assurance of proton range in future hybrid MR-proton therapy systems.”
The mechanisms underlying the observed effects are still to be unravelled. The most probable hypothesis, Schellhammer suggested, is that irradiating a liquid increases its temperature locally, creating a density difference in which the lighter heated water rises out of the imaging volume and creates a signal void. Further investigations are needed to test this hypothesis, she noted.
“I have demonstrated that proton beam range can be accurately verified with online MRI,” Schellhammer concluded. “However, today only at high doses and in fluid-filled phantoms. The method holds potential for dosimeter-free online quality assurance of MR-integrated proton therapy. Further research towards MR-based range proton beam verification is clearly justified.”