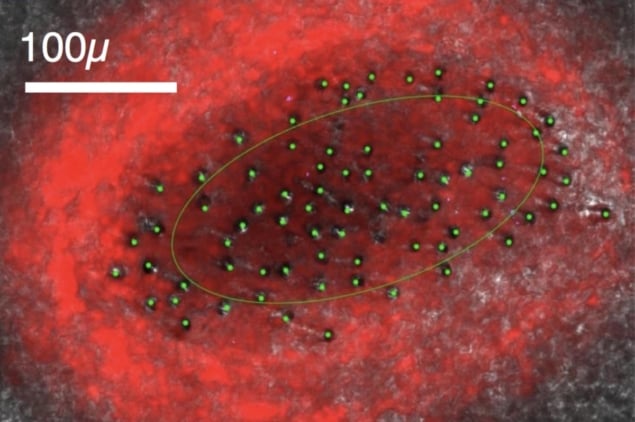
Optical fibres can be used to monitor neural activity by detecting the presence of a fluorescent protein. Researchers in the US have shown that bundles of hundreds or thousands of optical microfibres separate and spread after penetrating the brain, occupying a 3D volume of tissue. They also demonstrated that, when sampling density is high enough, source-separation techniques can be used to isolate the behaviour of individual neurons. The work will further the field of systems neuroscience by revealing how neural circuits relate to animal behaviour (Neurophotonics 10.1117/1.NPh.5.4.045009).
A relatively new way to monitor and influence brain activity, optical interfacing is used in transgenic animals whose neurons have been modified — either before birth or by an engineered virus introduced into the brain — to express a specific protein.
The protein used by Nathan Perkins and colleagues, at Boston University and the University of California, San Diego, changes shape when bound to calcium and, in this state, fluoresces green in response to blue light. Calcium-ion kinetics underlie one type of action potential in brain cells, so observing how these ions accumulate and dissipate gives an indication of neural activity.
Because of the scattering properties of brain tissue, direct illumination of target neurons deeper than about a millimetre is impossible to achieve with precision. Instead, excitation light and the resulting fluorescent signal are typically delivered and collected using implanted optical fibres.
The group previously demonstrated that bundles of several thousand optical microfibres — each just 8 μm across, or about the size of a neuron cell body — can be introduced to a depth of 4 mm without badly damaging the brain or provoking an immune response. As the tissue resists penetration by the microfibres, tiny mechanical forces cause their trajectories to diverge, spreading the individual tips over an area of about 1.5 mm2.
“The method can be readily extended down to 5 mm without any modification. Beyond that, a new challenge emerges, which is the flexibility of the fibres,” says Perkins. “This is not deep at all by human brain standards, but for mice and songbirds this allows us access to much of the brain.”
Simulated signals
Using stochastic simulations of the set-up, Perkins and colleagues modelled the fluorescence signal obtained for different neuron densities and microfibre counts, finding that the latter had the greatest influence on how many neurons could be detected simultaneously. At the lower end of the range, simulating a bundle composed of fewer than 200 microfibres, there was little overlap between excited regions, and each tip could detect the activity of a separate population of just two or three neurons.
As the microfibre count increased, the excitation light was delivered more uniformly throughout the modelled volume, causing more and more neurons to fluoresce at a level above the detection threshold. With a sufficient density of microfibres — and therefore illumination strength — the field-of-view of each tip expanded to encompass so many fluorescing neurons that any single cell would likely be recorded by more than one microfibre.
Under such a regime, the researchers showed, fluorescence signals picked up by separate microfibres can be correlated, enabling source separation techniques to be used to isolate the activity of individual neurons. The method is easiest to apply when signals are sparse (so the signature of a given neuron is not drowned out by background activity), and is especially effective with fluorescent indicators that peak sharply and fade quickly thereafter.
Until now, this degree of detail had been available only for superficial areas of the brain that can be imaged directly. At greater depths, researchers had observed individual neurons in isolation, or broad patterns of activity at the regional scale, but the long-term behaviour of a population of neurons was inaccessible. Understanding dynamics at this level is crucial to learning how the brain encodes information.
Music on the brain
An example of the sort of phenomenon that can be investigated with this approach has fascinated Perkins since the early days of his research: “Zebra finches are remarkable birds, where each male bird learns a unique song, inspired by that of their tutor (usually their father),” he explains. “They can then produce this song with amazing precision hundreds or even thousands of times per day for the rest of their life.”
The mystery, says Perkins, is that “neurons are able to produce this precise, stable behaviour even though they, like much of biology, are noisy and inconsistent”.
Previous experiments using optical recording for the brain’s surface, and surgically implanted electrodes at depth, showed that the patterns of activity behind the bird’s vocal performance remain stable even as the population of participating neurons varies. Although similar in form to the splayed optical microfibres used in the most recent research, the deep electrodes could not track the activity of individual neurons for longer than a day.
“Electrophysiological and optical interfacing offer very distinct trade-offs,” says Perkins. “Electrophysiological has much better time resolution and requires no fluorescent protein, but electrodes rarely can record from a specific cell for a long period of time. Optical recording has more longevity, allowing specific neurons to be tracked over many days, and the ability to target particular neural sub-populations.”

Ultramicroelectrodes deliver reliable neural recording
The optical method has another advantage in that it can control neural activity as well as monitor it. This is achieved by engineering neurons so that they express the proteins necessary to form light-gated ion channels — light-sensitive versions of the structures that regulate ion transport across cell membranes.
Because these ion channels and the fluorescent indicator proteins respond to different frequencies of light, the two processes could be made to occur simultaneously. “By being able to record what the neurons are doing in one context and re-activating the same neurons in a different context, it is possible to further understand their role in controlling behaviour,” says Perkins.