An upcoming change to SI units – due to be officially approved this month – will mark the end of a long journey from defining quantities in terms of objects to using precise, unchanging and universal constants of nature. Benjamin Skuse tells the story

On the outskirts of Paris, eight metres below ground in a climate-controlled vault, sits a 143-year-old platinum alloy cylinder. Standing just 39 mm tall, it has never been touched by human hands. Like a delicate Russian doll, the cylinder is caged inside three nested glass bells in a room that can be accessed only with three keys kept by three different people. Surrounding the mysterious object are “the witnesses”: six “identical” cylinders cast from the same platinum alloy.
Though preservation efforts rival those of the Turin Shroud, the cylinder is not a sacred religious object. It is the International Prototype Kilogram (IPK), the one and only true kilogram against which all others are measured. Housed in the Pavillon de Breteuil – home to the International Bureau of Weights and Measures (BIPM) – the IPK will soon lose its unique status and become a relic of a bygone age. It will then be as quaint as the International Prototype Metre (IPM) – a platinum alloy bar also housed at the BIPM – that served as the world’s official metre until 1960.
On 16 November 2018 metrologists and policy-makers from 60 countries around the world will gather at the General Conference on Weights and Measures (CGPM) in Versailles, France. Nothing unusual there, as the meeting convenes once every four years to discuss budgets and issues in metrology. But this meeting will be special. Member states will be voting on whether to adopt the most sweeping change to the International System of Units (Système International, or SI) since its inception in 1960. It is a change that will include new definitions of the kelvin, ampere and mole, but perhaps most significantly the kilogram.
Each member state will cast its one vote in a process that will be streamed live online. If the change is ratified (and all the signs are that it will be) the event will mark the end of basing units on objects – a practice dating back millennia. It will also finally fulfil a wish first voiced by James Clerk Maxwell, who predicted that measurement standards might somehow be defined by immutable constants of nature.
A solid foundation
Speaking at a meeting of the British Association for the Advancement of Science in Liverpool in 1870, Maxwell told delegates that “If…we wish to obtain standards of length, time and mass which shall be absolutely permanent, we must seek them not in the dimensions, or the motion, or the mass of our planet, but in the wavelength, the period of vibration, and the absolute mass of these imperishable and unalterable and perfectly similar molecules.”
With insiders seeing no hint of a negative outcome at the BIPM meeting this month, it is safe to assume that the resolution to reform the SI will pass unopposed and Maxwell’s desire for “absolutely permanent” standards will be realized. The new SI will then officially come into effect on 20 May 2019, precisely a dozen dozen (144) years after the first international treaty on units of measurement – the Metre Convention – which was signed on the same day in 1875. But why is it so important that units are based on constants of nature?
Units have been a staple of society since at least the time of the ancient Egyptians. They used different parts of the human body or objects in their environment as scales by which to measure things. Yet these standards could be wildly different from place to place. For natural philosophers in 17th- and 18th-century Europe, unit variability – particularly in length and mass – made it almost impossible to compare results for the same physical phenomenon if it had been measured in different places.
Various attempts were made at creating a universal measure and in 1799 France introduced the metric system, based on two units – the metre and the kilogram. Known as the Metre of the Archives and the Kilogram of the Archives, these two platinum artefacts were stored at the Archives Nationales in Paris to legally and practically define the units. These standards stood for 90 years until they were replaced by the IPM and IPK, which were physically harder and better designed.
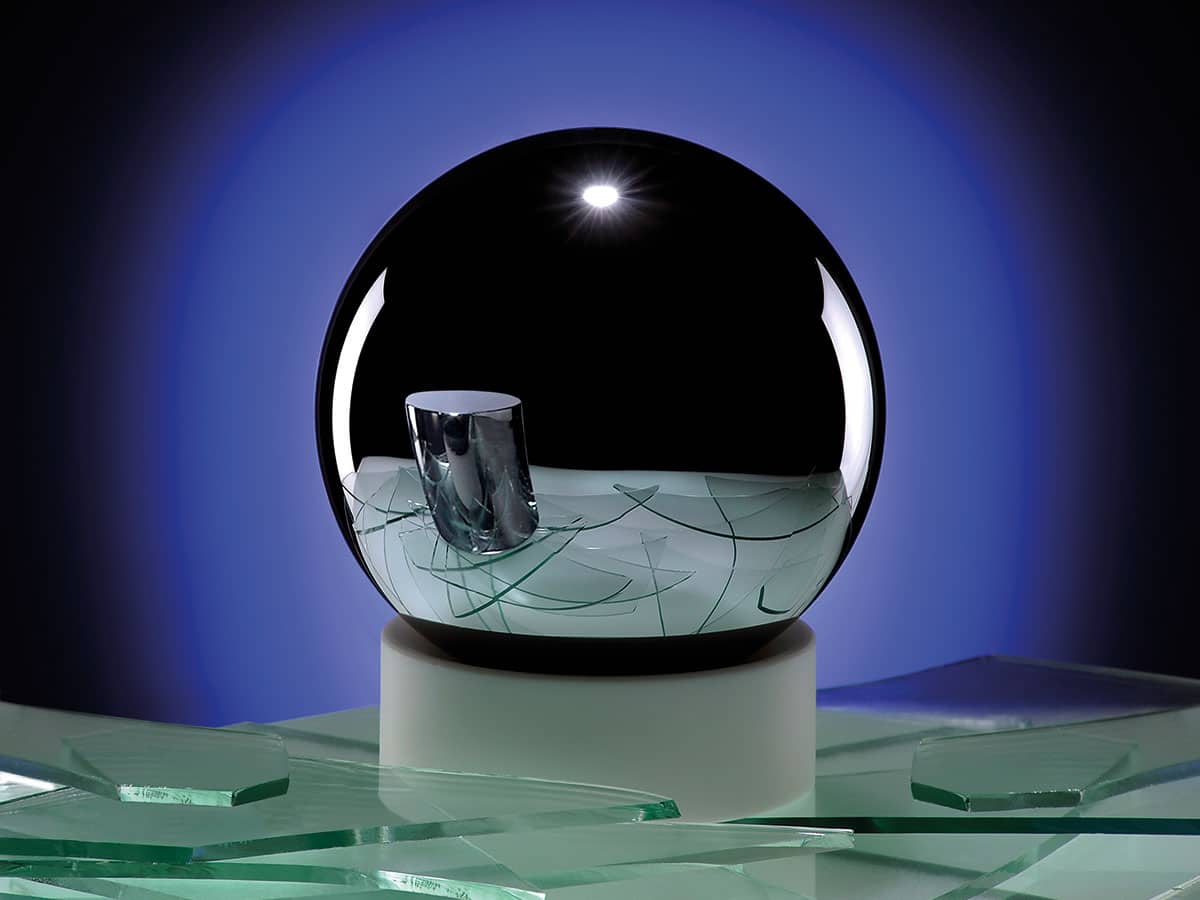
Universal thinking
SI units have become entwined in science. From the energy-defining joule to the katal for measuring catalytic activity, all 29 named SI units can be defined by some combination of just seven base units: the second, metre, kilogram, ampere, kelvin, mole and candela. But as science became ever more precise in the 20th century, a new problem reared its head. Any unit based on something – an object, experiment or phenomenon – that is not universal will be unstable.
Consider the second. It is historically linked to the revolution of the Earth, which is defined as taking 24 hours, where an hour is 60 minutes, and a minute is 60 seconds. But what happens if the Earth starts to rotate more slowly, as it is doing albeit ever so slightly? A day will be longer, meaning a second will be longer in real terms too. It means a car registering 30 km/h will actually be travelling a little slower, a 30 W bulb will be a little dimmer and, even more absurdly, the universe will be expanding at a different rate.
If, however, the notion and duration of a second are kept but the Earth’s rotation is removed from the definition and replaced with something that never changes wherever and whenever it is measured in the universe, the second becomes stable. This was done in 1967, when the second was redefined as 9,192,631,770 times the period of the radiation corresponding to the transition between the two hyperfine levels of the ground state of the caesium-133 atom, Δν (see “A brief history of time-keeping” by Helen Margolis).
Later, in 1983, the metre was also redefined, as the length of the path travelled by light in vacuum during a time interval of 1/299,792,458 seconds. The exquisite precision with which scientists have since been able to measure time and distance has benefited society, not least by leading to satellite-based positioning systems, notably GPS.
Planck to the rescue
Despite having served society well for 143 years, defining the kilogram in terms of a single object is an inherently unstable notion. That’s because if the IPK becomes lighter or heavier, even by a tiny amount, the mass of the universe expressed in kilograms changes too – a mad proposition. Rather worryingly, the IPK has been changing. When metrologists measured it in 1988–1991, the IPK had a mass around 50 μg less on average than the six witnesses. By definition, this means that the witnesses had gained a tiny amount of mass somehow, perhaps by absorbing air molecules. But more probable – given that many national copies of the kilogram also appeared to be gaining mass – is that the IPK had lost mass. Or perhaps they had all gained or lost mass, just at different rates.
Metrologists saw no further drift between the IPK and the witnesses from 1991 until 2014, the last time measurements were made. But the fact there was no drift didn’t mean the mass of the IPK or the witnesses had not changed. They may simply have been losing or gaining mass in tandem. And that’s the problem: there is no way of telling because mass is always calibrated against the IPK.
“With the revised SI, we won’t have to worry about this stuff,” explains Richard Davis, a former head of the BIPM’s mass division who is now a consultant to the bureau. Instead of being defined by the mass of a cylinder of metal, in the new SI the kilogram will be based on a fundamental constant of quantum physics: the Planck constant.
Named after Max Planck, who developed the idea that energy comes in small packets called quanta, the Planck constant, h, relates the energy of one quantum of electromagnetic radiation to its frequency by the famous formula E = hν. The Planck constant is in turn linked to mass via Einstein’s E = mc2. Currently, h has a measured value of approximately 6.62607 × 10–34 m2 kg s–1, but metrologists now want to fix its value in stone, with the kilogram defined in terms of this value.
It’ll therefore be goodbye to the IPK, which is a physically unstable object, and goodbye to uncertainty in the value of Planck’s constant. “After the redefinition, the unwavering Planck’s constant is fixed to a value, while the uncertainty is more appropriately shunted to the mass of the IPK,” says Stephan Schlamminger, a metrologist from the National Institute of Standards and Technology, US. “And with a fixed Plank constant, better devices will be able to realize a kilogram more and more precisely.”
Watt do you mean
To start off on the right foot, it is important that the value at which Planck’s constant is fixed is measured as precisely as currently possible. This responsibility rests on measurements from two very different types of experiments. The first of these is called a Kibble balance, formerly called a watt balance but now renamed in honour of its inventor Bryan Kibble from the UK’s National Physical Laboratory, who died in 2016. Currently, only France, Canada and the US have Kibble balances capable of making the measurements needed to fix the Planck constant. However, many others are working on building balances of their own. Like a hi-tech set of scales, the Kibble balance uses electromagnetic forces provided by a coil of wire immersed in a magnetic field to balance a kilogram mass. The equipment lets metrologists take accurate values of current and voltage, from which the Planck constant can be derived (see box below).
The Kibble balance
What is it? The Kibble (or watt) balance consists of a circular, horizontal coil of wire of length, L, hung from one arm of a balance. The coil is placed in a strong magnetic field, B, and an electric current, I, is passed through it generating a force, F = BIL, that can be adjusted to equal the weight of a mass placed on the same arm of the balance (mg). The mass is then given by m = BIL/g.
What’s the problem? Although I can be measured accurately, it’s hard to do the same for B and L.
So what’s the solution? Metrologists remove the mass and move the coil at speed u in the magnetic field to generate a voltage V = BLu. The device is termed a watt balance because, by rearranging the two equations, electrical power (VI) is balanced by mechanical power (mgu). In other words, m = VI/gu. As u is easy to measure and g (the acceleration due to gravity) is well known, the problems with measuring B and L have disappeared.
But what’s the link with the Planck constant, h? That’s the clever bit. The current is determined by passing it through a resistor and using the Josephson effect to measure the resulting drop in voltage. This effect describes the fact that if two superconductors are separated by a thin insulator, pairs of electrons in each layer couple so that microwave radiation of frequency, f, creates a voltage across the layer of V = hf/2e, where e is the charge on the electron. The resistance of the resistor can be measured because the electron flow in 2D systems at ultralow temperatures is quantized, with the conductivity increasing in multiples of e2/h.
So why is this good for metrology? Until now, a Kibble balance measured h in SI units. But when the definition of the kilogram is changed, the numerical value of h will be fixed in stone, allowing anyone to use the balance to measure mass with exquisite precision.
The second way of measuring h is called X-ray crystal density (XRCD) or the Avogadro experiment. It involves a uniform crystal of silicon-28 atoms that has been machined into almost a perfectly round 1 kg sphere. Using optical interferometry, metrologists first calculate the overall diameter – and hence the volume – of the sphere. Then, by combining optical interferometry with X-ray analysis, they can calculate the spacing between atoms, the volume each occupies, and thus the total number of atoms in the sphere. Finally, by weighing the sphere, they can determine the Avogadro constant. This approach defines how many atoms or molecules there are in one mole of a substance – a quantity quite different to mass, which will now define the mole itself. An equation from atomic physics that links the Avogadro and Planck constants then allows a precise value of the latter to be captured.
Laboratories across the world have used these two different techniques to measure the Planck constant with extraordinary precision to give a final, agreed value of 6.626,070,150 × 10–34 kg m2 s–1, with a relative uncertainty of only 10 parts per billion (2018 Metrologia 55 L13). As for the Avogadro constant, it will be fixed at 6.022,140,76 × 1023 mol–1. And once the Planck and Avogadro constants are fixed, the complex experiments from which they were derived can be used as standards for measuring a kilogram and a mole.
Practical impact
The easiest way to spell out how this will work is to consider the Kibble balance. Until now, it has been used to measure accurate values of current and voltage that are then plugged into equations to yield the Planck constant. In the future, the Planck constant will be a fixed value and those same measurements will instead yield the mass on the balance. In other words, anyone with access to a Kibble balance can realize a perfect kilogram. The same principle will apply to the ampere and kelvin too, which will in future be given in terms of the charge on an electron, e, and the Boltzmann constant, k, respectively. Equipment designed to precisely measure these fundamental constants will now be turned on their heads to accurately realize the ampere and kelvin units (see box below). As for the metre, second and candela, their definitions will be tweaked but will remain effectively unchanged.
Out with the old, in with the new
SI mass unit: kilogram
Old: The kilogram is equal to the mass of the International Prototype Kilogram.
New: The kilogram (kg) is defined by taking the fixed numerical value of the Planck constant h to be 6.626,070,150 × 10–34 when expressed in the unit J s, which is equal to kg m2 s—1, where the metre and the second are defined in terms of c and ∆ν.
Translation: The kilogram will be defined in terms of Planck’s constant instead of the mass of a cylinder of metal called the International Prototype Kilogram.
SI electric current unit: ampere
Old: The ampere is that constant current which, if maintained in two straight parallel conductors of infinite length, of negligible circular cross-section, and placed 1 m apart in vacuum, would produce between these conductors a force equal to 2 × 10–7 N per metre of length.
New: The ampere (A) is defined by taking the fixed numerical value of the elementary charge e to be 1.602,176,634 × 10–19 when expressed in coulombs, which is equal to A s, where the second is defined in terms of ∆ν.
Translation: The ampere will be defined in terms of how many elementary electrical charges pass per second instead of by an imaginary and impossible experiment involving the force between two infinite parallel, current-carrying wires.
SI amount of substance unit: mole
Old: The mole is the amount of substance of a system that contains as many elementary entities as there are atoms in 0.012 kg of carbon-12.
New: The mole (mol) contains exactly 6.022,140,76 × 1023 elementary entities. This number is the fixed numerical value of the Avogadro constant, NA, when expressed in the unit mol–1 and is called the Avogadro number.
Translation: The mole will be defined in terms of a specific number of atoms or molecules, rather than by a quantity intimately connected to measuring the mass of a sample.
SI thermodynamic temperature unit: kelvin
Old: The kelvin, unit of thermodynamic temperature, is the fraction 1/273.16 of the thermodynamic temperature of the triple point of water.
New: The kelvin (K) is defined by taking the fixed numerical value of the Boltzmann constant k to be 1.380,649 × 10—23 when expressed in the unit J K1, which is equal to kg m2 s—2 K1, where the kilogram, metre and second are defined in terms of h, c and ∆ν.
Translation: The kelvin will be defined through the constant relating thermodynamic temperature to energy (Boltzmann’s constant), instead of by the point at which water coexists as a liquid, gas and solid.
Outside metrology, the new SI will have little immediate practical consequence, and will go unnoticed by most people. After all, the units may be defined differently, but the goal is always to keep their size the same. Yet defining the kilogram, kelvin, ampere and mole in entirely new ways based on constants of nature makes them invariant, accessible and practical. Therefore, scientists will be able to measure them at any place or time, and on any scale.
“For the first time, we will be able to measure tiny and huge quantities with the same very high precision because the fundamental constants don’t care about a scale,” adds Schlamminger. This is important. Before the metre was redefined, length could be measured only very precisely around a metre. But since its redefinition, high-precision applications like microelectronics have benefited enormously from the accuracy with which they can measure distance at tiny scales.
Similarly, the new kilogram will allow a kilogram, gram and milligram to be measured with identical precision, even to the point that atomic masses will be measured in kilograms. As long as there is an experimental connection to the Planck constant, mass will be able to be measured. Therefore, metrologists are racing to build tabletop Kibble balances and new devices that measure mass exactly at scales big and small. So the new mass unit and SI units generally are finally fit for the 21st century, and will remain so long into the future. As Schlamminger aptly concludes: “The new SI is a construction of beauty and logic.”
- For more on the new SI definitions, check out the free-to-read Physics World Discovery ebook Redefining the Kilogram and Other SI Units by Stephan Schlamminger at www.physicsworlddiscovery.org