Every few years the eastern part of the Pacific Ocean warms up around the equator and plays havoc with the climate on several continents. Why does this happen?
The past two years have witnessed the strongest El Niño this century. Indeed, the event received so much media coverage that the phrase “El Niño” is now part of our everyday vocabulary. The impact of the 1997/8 El Niño was felt in many parts of the world: floods and warm weather led to a failed anchovy season in Peru; torrential downpours and mud slides besieged southern California; droughts caused uncontrollable forest fires in Indonesia; coral in the Pacific Ocean was bleached by hotter than average water; and shipping through the Panama Canal was restricted by below-average rainfall.
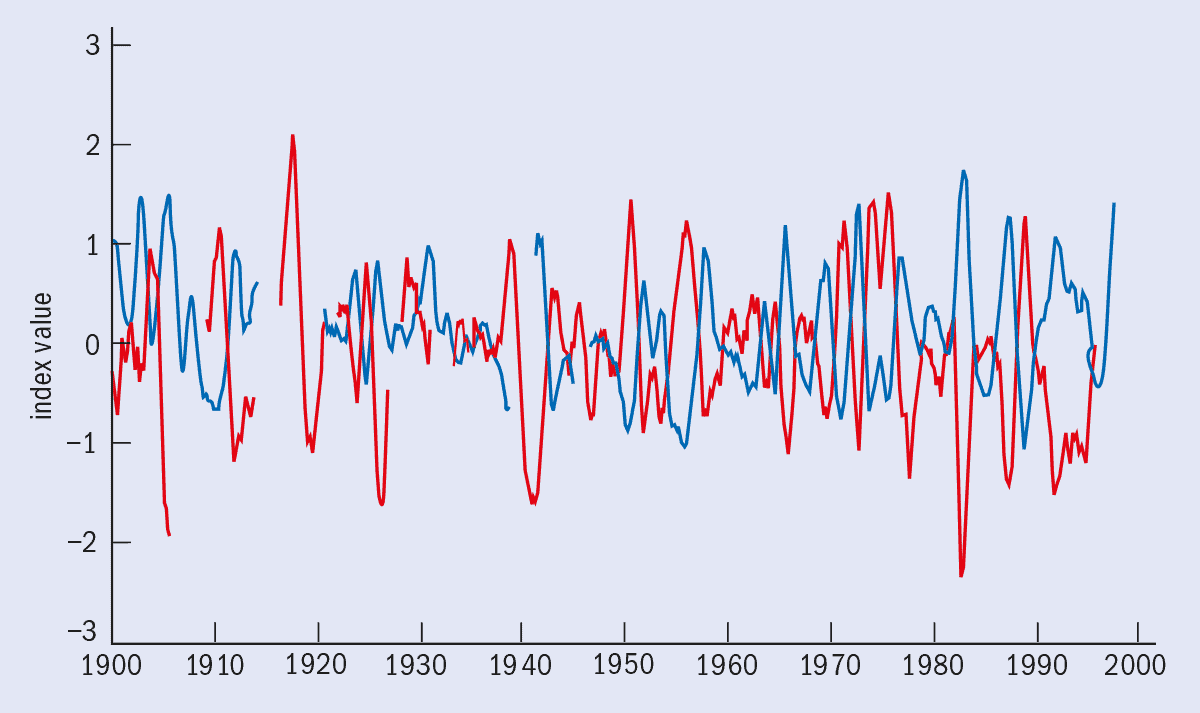
Long before its global impacts were known, Peruvian fisherman had given the name El Niño to a warm southward current that normally appeared in the first months of each year. Every few years, however, the return of the warm current started earlier, around Christmas, and the fisherman called it El Niño (meaning “the Christ child”).
During the 1960s and 70s, oceanographers used El Niño to refer to an anomalous large-scale warming in the equatorial eastern and central Pacific. At about the same time, scientists began to realize that this warming was intimately linked to an atmospheric phenomenon – the Southern Oscillation – discovered by Sir Gilbert Walker in 1923. The Southern Oscillation is, in simple terms, a “seesaw” of atmospheric pressure between the Pacific and Indian oceans. The clearest sign of the oscillation is the inverse relationship between the air pressure measured at two sites: Darwin, Australia, in the Indian Ocean and the island of Tahiti in the South Pacific. When the air pressure rises in Darwin, it falls in Tahiti, and vice versa.
By the early 1980s it was evident that El Niño and the Southern Oscillation were related, and scientists coined the acronym ENSO to describe this large-scale, interannual climate phenomenon. Various indices have been used to characterize ENSO. The Southern Oscillation Index (SOI) is the difference in sea-level pressure measured at Darwin and Tahiti, while the Cold Tongue (CT) Index measures how much the average sea-surface temperature in the central and eastern equatorial Pacific varies from the annual cycle (figure 1). It is evident that the two indices are anti-correlated, so that a negative SOI is usually accompanied by an anomalously warm ocean – El Niño.

Although ENSO is inherently caused by interactions between the atmosphere and the ocean in the tropical Pacific, it is responsible for changes in the global climate system that are especially evident throughout the western hemisphere (figure 2). The anomalies that can be seen in the northern Pacific have been shown to be “teleconnected” from the tropics via a large-scale redistribution of vorticity in the atmosphere.
Since recognizing the importance of ocean-atmosphere interactions to ENSO, remarkable progress has been made towards a comprehensive understanding of the phenomenon. An ENSO observing system has been established over the past 10-15 years and we can now observe the state of the upper tropical Pacific Ocean in real time (figure 3). We have also built accurate climatologies of the surface winds and the upper ocean (both its thermal structure and currents), and developed techniques to combine data from sparse observations with simulations, producing historical data sets that are spatially complete and consistent with the laws of physics. Finally, we have constructed coupled ocean-atmosphere models that are capable of simulating many aspects of ENSO.
All this progress means that we are gradually starting to understand the nature of the annual, year-to-year and long-term variability of the Pacific. We have begun to use data from the observing system for operational seasonal-to-interannual climate forecasting, and have demonstrated that the state of the coupled ocean-atmosphere system in the tropics can be predicted several seasons in advance.
Characteristics of ENSO events
Observations show that ENSO is a highly variable phenomenon: events last about 12-18 months and the time between events ranges from two to seven years. The strength also varies greatly from event to event (figure 1). Further analysis indicates that for most warm ENSO events, the maximum warming in the eastern equatorial Pacific occurs in December and January. The following features also seem to be common to most ENSO events.
(a) Quasi-stationary anomalies in the sea-surface temperature (SST) in the eastern and central Pacific.
(b) A relaxation of the trade winds – easterly winds in the tropical Pacific – associated with positive SST anomalies at the onset of the event.
(c) A deepening in the east and a shallowing in the west of the thermocline along the equator. (The thermocline is the zone at the top of the ocean in which the water temperature decreases rapidly with depth.)
(d) Prior to the peak of the ENSO event, the anomalously deep thermocline in the eastern/central Pacific begins to return to normal values.
(e) The trade winds in the far western Pacific increase one or two seasons before the onset of an event.
Despite these common traits, the detailed features of any single ENSO event can vary considerably, including when and where the initial warming starts. For example, sea-surface temperatures near Peru increased by more than 5 ºC during the 1997/8 event. In contrast, in the 1986/7 event the warming extended only as far the mid-Pacific and the maximum temperature was a modest 1 ºC above normal.
Events also vary on longer timescales. During the 1930s and 40s, for example, ENSO events were not as frequent or as severe as those in the past three decades. This irregularity reflects the complexity of the coupled ocean-atmosphere system and hints at the difficulties in predicting ENSO. Therefore, understanding the irregularity of ENSO is a major area of endeavour in climate research.
Understanding ENSO: early ideas
Although it was discovered by Walker in 1920s, the first major breakthrough in understanding the Southern Oscillation and its oceanic counterpart, El Niño, occurred in the 1960s when the Norwegian meteorologist Jacob Bjerknes made two very important discoveries.
First, he noted that the Southern Oscillation is a perturbation to what he called the Walker circulation – a thermally driven east-to-west circulation across the equatorial Pacific. In the Walker circulation, moisture-laden air converges onto the warmest regions of the world’s oceans – the western equatorial Pacific – where it rises and the moisture condenses. This leads to widespread cloudiness and heavy precipitation. In the eastern equatorial Pacific, where the surface water is relatively cold, dry air descends from the upper troposphere and prevents substantial rainfall. In the Walker circulation these motions – rising in the west, sinking in the east – are connected through easterly trade winds near the surface and a westerly wind aloft.

The Southern Oscillation is associated with fluctuations in the intensity and position of the rising moist air, and hence with changes in the prevailing trade winds over the tropical Pacific. Bjerknes was the first to recognize the coupling between changes in the oceanic and atmospheric circulations during ENSO. Based on the observations, he reasoned that the east-to-west increase in the sea-surface temperature in the tropical Pacific and the overlying trade winds were intimately coupled, and that the temperature difference along the equator reinforced the strength of the trade winds.
In turn, the easterly wind stress acting on the ocean surface causes the thermocline to rise and the cold subsurface water to upwell in the east. The trade winds and the associated equatorial upwelling maintain the climatological distribution in the sea-surface temperature: a warm pool in the west and a cold “tongue” in the east. However, a modest change in either the equatorial SST or the trade winds can trigger a chain reaction because the ocean and atmosphere are so closely coupled. For instance, if the equatorial trade winds get weaker, the equatorial upwelling will decrease and the thermocline will get deeper, leading to warming in the east and even weaker trade winds (figure 4a). This positive-feedback mechanism, often known as the Bjerknes hypothesis, is believed to be one of the two key ingredients that are responsible for ENSO events.
Bjerknes’ hypothesis laid the foundation for much of the subsequent progress in understanding and modelling ENSO. However, his ideas posed a dilemma: what stops the warming (the positive feedback) in the eastern Pacific? Why do El Niño events typically last only 12-18 months? And why, after about a year, do they usually shut down rapidly and switch to cold conditions?
The middle ages
After Bjerknes’ initial breakthroughs there followed significant theoretical advances in our understanding of how the equatorial ocean responds to wind stress, and of how the atmosphere responds to anomalies in the sea-surface temperature. By the end of the 1960s it was clear that the equatorial region is special: the Coriolis force (which results from the Earth spinning) vanishes at the equator and creates a waveguide in which a variety of waves are trapped to within a few degrees of the equator in the ocean (and a few tens of degrees in the atmosphere). These waves play an important role in determining how the tropical ocean and atmosphere respond to various changes in the surface forcing.
Two types of waves, the equatorial Kelvin and Rossby waves, are of particular importance. Kelvin waves are special gravity waves that propagate eastward with a speed of approximately 2-3 ms-1 in the ocean and have their maximum amplitude at the equator. Rossby waves, which are driven by the variation of the Coriolis force with latitude, propagate westward at about 0.6-0.8 ms-1 near the equator. The oceanic Kelvin and Rossby waves carry energy and momentum received from the wind stress at the ocean surface. They also provide the oceanic “memory” that is so important to year-to-year variability and ENSO.
Rossby and Kelvin waves also exist in the atmosphere, but these move much faster than their oceanic counterparts. This difference in speed means that the tropical atmosphere adjusts to changes in the SST much quicker (10 days or less) than the equatorial ocean responds to changes in wind stress (about six months). The short adjustment time of the atmosphere allows the assumption that the atmosphere is in statistical equilibrium with the SST on timescales longer than a few months. Thus, the long-term memory of the climate system primarily resides in the ocean.
Modelling El Nino and the Southern Oscillation
Advances in modelling the ocean and atmosphere circulations have contributed significantly to our understanding of ENSO. The truly crucial advance was the advent of coupled atmosphere-ocean models. Broadly speaking, the models that have been used to study ENSO can be divided into two types: statistical models and dynamical models based on the laws of physics. Our understanding of ENSO has undoubtedly benefited from both modelling approaches.
Dynamical models can be further divided into three groups. Intermediate coupled models (ICMs) consist of a simple atmosphere model coupled to a simple ocean model. The atmosphere is usually constructed so that winds in the atmospheric boundary layer adjust instantaneously to changes in the SST. The ocean is approximated as immiscible layers of hot and cold water, with changes in the depth of the thermocline between the layers being determined by the conservation laws for mass and momentum. Hybrid coupled models (HCMs) consist of a similar atmosphere coupled to a general circulation model that solves the three-dimensional ocean circulation along with temperature, salinity and other chemical tracers according to the conservation laws for mass and momentum.
Coupled general circulation models (CGCMs) combine general circulation models for both the ocean and the atmosphere. The most complex of the models, CGCMs require extensive computational resources.
In all coupled models, the ocean component must vary with time to allow for the Rossby and Kelvin waves that provide the memory for ENSO. The major difference between simple and general circulation models is in the ability to simulate vertical structure and nonlinear processes in the oceans.
The atmospheric components of the models, on the other hand, differ fundamentally. The simple models capture only a portion of the atmospheric variability that is driven by the SST (sometimes called the “signal”) and ignore completely the portion that is determined by internal atmospheric dynamics, such as hydrodynamic instability and turbulence (the “noise”). In contrast, general circulation models simulate a full spectrum of atmospheric variability, including both the signal and the noise.
Many of the coupled models produce ENSO-like interannual variability through ocean-atmosphere interactions, providing further support to the notion that ENSO is of the coupled atmosphere-ocean system phenomena. However, the simulated interannual variability exhibits a rich variety of behaviour in, for example, the distribution and evolution of temperature anomalies, or the strength and the dominant oscillation period of ENSO cycles. Nevertheless, coupled ocean-atmosphere models have been used extensively to explore the physics of ENSO and to simulate events that evolve via the delayed-oscillator physics.
Meanwhile, simple models (ICMs and HCMs) have been used to explore the importance of nonlinear dynamics in ENSO and identify different dynamical regimes of the coupled system. On the one hand, extensive numerical experiments have shown that the irregularity of ENSO can be generated by a low-order chaotic process due to nonlinear interactions between the annual cycle and interannual oscillation of the coupled system. On the other hand, these same models, when first stabilized by tuning various parameters and then forced by stochastic noise, support ENSO-like variability where self-sustaining oscillations are not possible. Although the character of any natural stochastic noise is unknown at the moment, it is likely that stochastic atmospheric processes provide a major source of ENSO irregularity in nature.
The renaissance
The free, oceanic Kelvin and Rossby waves can be strongly modified by the air-sea coupling. Indeed, the coupling between the ocean and atmosphere generates new wave “modes” with characteristics that depend on the physical processes that control the dynamical and thermodynamical adjustment of the ocean. There are two key timescales for ENSO: one associated with the dynamical adjustment of the equatorial ocean, and the other associated with the changes in the SST due to air-sea coupling.
Two extreme limits are interesting: when the dynamical adjustment of the ocean is either fast or slow compared with the changes in sea-surface temperature. In the fast dynamical limit, the behaviour of the coupled ocean-atmosphere system depends critically on the time evolution of the SST, but is less influenced by the ocean-wave dynamics. In the limit of slow dynamics, the modes that are supported in the coupled ocean-atmosphere system are very similar to free, equatorial ocean waves. These waves provide the memory that allows for an oscillation.
In nature, however, the timescale of the ocean adjustment is comparable with that associated with SST changes due to the air-sea coupling, and the response of the coupled system has a decidedly mixed flavour that is described by a “delayed-oscillator mode”. The behaviour of the ENSO mode can be described by a differential-delay equation for the sea-surface temperature anomaly, T, in the eastern equatorial Pacific (the boxed region in figure 4a) dT /dt = cT – bT (t – t) where t is time and t is the timescale associated with the dynamical adjustment of the ocean basin. The right-hand side of the equation consists of two parts: cT represents the positive feedback proposed by Bjerknes, while bT (t – t) is a delayed negative feedback representing adjustment processes in the ocean (figure 4b).

The delayed negative feedback proceeds as follows: although the westerly wind anomaly (in response to warming in the east) deepens the thermocline along the equator, it also causes the thermocline to rise in regions away from the equator (between about 3º and 8º north and south). These off-equator thermocline anomalies propagate stealthily (with respect to the sea-surface temperature) to the west as Rossby wave packets, but otherwise have little effect on the SST.
When the Rossby signals reach the Indonesian archipelago, they are reflected back as Kelvin waves along the equator and cause the thermocline to rise back towards the surface. In the western Pacific, the thermocline is already so deep that the local SST is not affected. In the east, however, the mean upwelling is strong and the thermocline is, on average, much closer to the surface (even during a warm ENSO event). Hence, as the Kelvin signal moves into the eastern Pacific, the thermocline moves even closer to the surface. It is the resultant surface cooling that is responsible for the shutdown of the local Bjerknes positive feedback, and hence the termination of the warm ENSO event.
There is a gap of about six months between the creation of the Rossby waves in the central Pacific and the return of the Kelvin wave to the region. This timescale, t, is comparable with that associated with the local Bjerknes mechanism. It also provides the “memory” of the coupled system that is essential for the shutdown of warm and cold ENSO events, and for the typical transition from warm into cold events – often called La Niña. The delayed-oscillator theory advances our understanding of ENSO because it offers an explanation for the evolution and duration of ENSO events (about one year) and for the tendency (at times) for the system to have perpetual turnarounds from warm to cold states and back again, which the Bjerknes hypothesis cannot explain.
The future

Is the delayed oscillator the real mechanism for ENSO? Observations are remarkably consistent with the positive-feedback mechanism during the development of an ENSO event. There is also evidence to suggest that the delayed-adjustment processes due to Rossby and Kelvin waves contribute to the termination of a warm event. This year’s ENSO event evolved in a way that was entirely consistent with the delayed-oscillator hypothesis (figure 5).
However, there are inconsistencies between observations and theory during the onset of events. For example, the strengthening of the trade winds that sometimes precedes the event is not a feature of the delayed-oscillator theory. (Since the delayed-oscillator theory views ENSO as a self-sustained regular cycle it is meaningless to discuss whether the cause of ENSO resides in the atmosphere or in the oceans – any small perturbation to either can trigger oscillations in the combined system.)
Some scientists have also questioned the idea that the coupled atmosphere-ocean system is unstable: they argue that the tropical Pacific is a stable system and that the ENSO variability can be viewed as a response of such a system to stochastic processes in the atmosphere. Simulations have suggested that ENSO-like variability can be generated by external random forcing in a globally stable dynamical regime (in which a self-sustained ENSO-like oscillation is not possible). In these models the development of a warm event is interpreted as a constructive interference of stable non-normal modes in the system. An attractive feature of the stochastic theory is that it offers a natural explanation in terms of noise to the irregular behaviour of ENSO variability.
The other competing hypotheses for the ENSO irregularity include nonlinear interactions between ENSO and the seasonal cycle, and the inherent nonlinearity of the coupled system.
Predicting ENSO
Among the greatest achievements in our understanding of ENSO is the establishment of a theoretical foundation for the prediction of short-term climate variability. The quasi-periodic nature of ENSO implies a good deal of predictability. Unlike the weather, which is known to be unpredictable beyond approximately two weeks due to the chaotic nature of the atmosphere, the interannual variability of sea-surface temperatures associated with ENSO can be predicted several seasons – perhaps more than a year – in advance. And because tropical sea-surface temperatures serve as an important boundary condition on the global atmosphere, the ability to predict these offers the possibility of making forecasts of ENSO-related atmospheric anomalies.
The first successful forecast of ENSO was made using an intermediate coupled model developed by Mark Cane and Steve Zebiak at the Lamont-Doherty Geological Observatory of Columbia University in the US. The model successfully predicted the onset of the 1986/7 El Niño one year in advance. Since then, the field of ENSO prediction has grown and there is now a variety of models that have demonstrated skill in forecasting the SST in the central and eastern equatorial Pacific up to a year in advance (see on models). ENSO forecasts are now available in the monthly Climate Diagnostics Bulletin and the quarterly Experimental Long-Lead Forecast Bulletin.
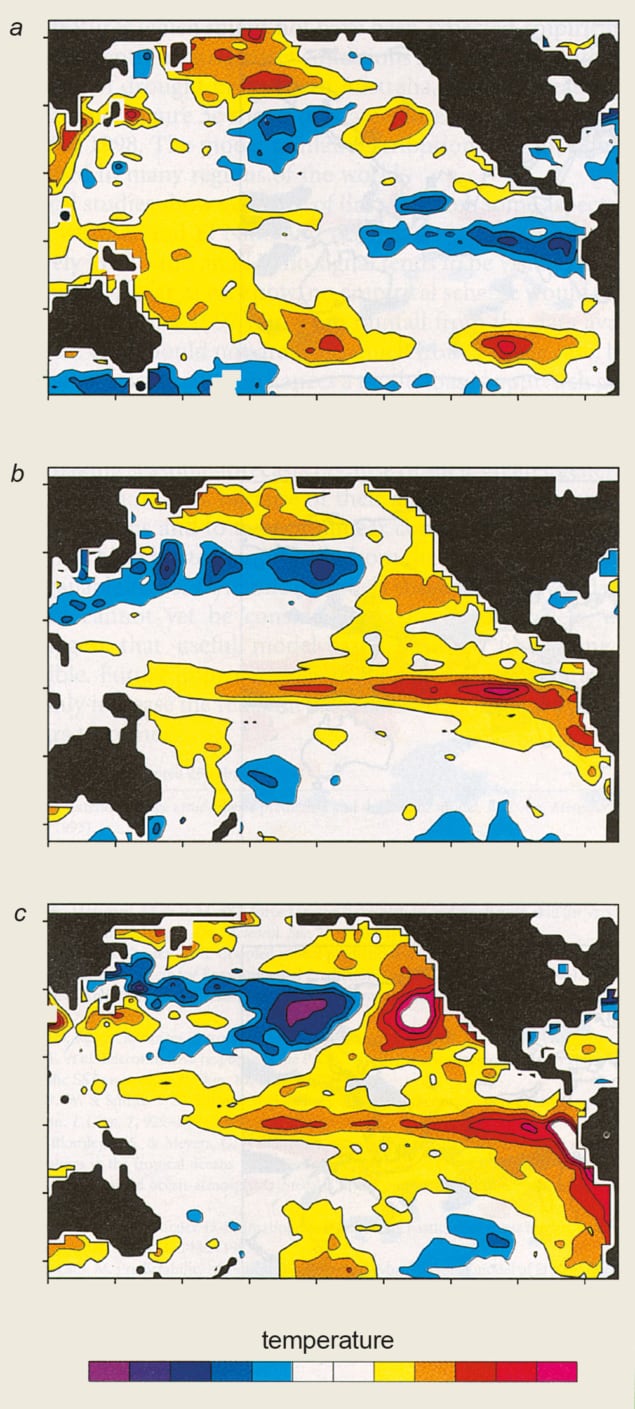
Generally speaking, the statistical models are better than dynamical models for short prediction times (less than six months), but less accurate for longer lead times. This may be partly due to the different initialization procedures used by the models. In an effort to improve the procedures used by dynamical forecast models, various schemes have been developed to use more real data in the simulations. This combination of “data assimilation” and global coupled general circulation models for short-term climate prediction has been implemented in several meteorological centres around the world, including the US National Center for Environment Prediction (NCEP) in Camp Spring, Maryland, and the European Centre for Medium-Range Weather Forecasts (ECMWF) in Reading in the UK. Both the NCEP and the ECMWF systems successfully predicted the onset of 1997/8 El Niño six months in advance (figure 6).
In addition to predicting various winter rainfall patterns commonly associated with ENSO (wet conditions in California, Florida, Uruguay and East Africa, and dry conditions in the Amazon region and southern Africa), the ECMWF model also predicted unexpected weather patterns such as wet conditions in India and southeast China, the absence of drought in northern Australia and the unusually wet climate in western Europe this past winter.
But despite the recent success in predicting the 1997/8 ENSO event, many fundamental questions concerning ENSO predictability remain. What are the dominant physical processes that limit the predictability of ENSO? Are the stochastic processes that are important for the irregularity of ENSO internal to the atmosphere or the ocean? Or are they due to the inherent nonlinearity of the coupled system? Are external forcing and tropical-extratropical interactions responsible for the decadal-to-century modulation of ENSO?
Studies have shown that the skill of many ENSO forecast models drop off considerably when they try to make predictions beyond spring in the northern hemisphere, regardless of when the predictions are made. The dynamical processes that contribute to this “spring predictability barrier” are not clear and we do not know if this is an intrinsic barrier to climate predictions in the tropics. Furthermore, the severity of the barrier appears to undergo a decadal modulation: for instance, the barrier appeared to be stronger in the 1960s and 70s than in the 80s. This decadal modulation may have an effect on the accuracy of model. Finally, most models failed to predict the event that occurred during 1990 to 1994.
Currently, we do not understand why some ENSO events appear to be more predictable than others. Some investigators have speculated that certain events, such as the 1990/4 warming, may be manifestations of a decadal mode in the coupled system with physics that is considerably different to ENSO dynamics. Others believe that stochastic processes play the most important role in determining the predictability of ENSO.
Looking ahead
Much has been accomplished in the past decade in observing, understanding and predicting ENSO. A comprehensive ENSO observing system is in place; models have been developed that simulate ENSO and predict tropical Pacific sea-surface temperature anomalies at lead times of up to a year; and the prospects for seasonal forecasts of short-term climate variability are improving rapidly. Currently, one of the major research activities is to assess how much of this tropical Pacific predictability can be translated into useful climate predictions for the entire globe on seasonal-to-interannual timescales.
Although the impact of ENSO is global, not all anomalous weather patterns are linked to it. In the mid-latitudes, in particular, its effect is small compared with internally generated atmospheric variability. It has also become clear that there are other modes of climate variability – such as monsoons – that have a profound influence on regional climate. Many of these patterns interact with each other (and with ENSO). For example, observational evidence suggests that ENSO is responsible for a portion of the variability of the Asian-Australian Monsoon, and that the temperature variability in the Pacific and Atlantic oceans influences the American Monsoon. Studies also suggest that the variability of the tropical Atlantic basin might have a large impact on the climate over the Americas and Africa. Understanding the multi-basin interactions among the tropical oceans and neighbouring land processes will be a major challenge for future research.
Variability in extratropical climates has also recently received considerable attention. For example, the North Pacific Decadal Oscillation – a strong SST signal that varies on 20-30 year timescale – has a footprint in the north Pacific that is very similar to that due to ENSO (see figure 2). The variability of the North Atlantic Oscillation (NAO) also has a strong influence on the European climate. These mid-latitude climate anomalies are not well understood, although it is generally believed that internal atmospheric variability is important on decadal and longer timescales. Exploration of the basic mechanisms and predictability of decadal climate variability will be an exciting area of future research.
Last, but not least, the effect of global warming due to increases in greenhouse gases and other anthropogenic changes on ENSO is largely unknown. Although coupled general circulation models (GCMs) have been used to study the global-warming scenario, all but one of these models have coarse resolution in the tropics and do not give a realistic representation of ENSO variability. To truly understand the relationship between anthropogenic climate change and natural climate variability, coupled GCMs must achieve accurate simulations of both the natural variability and the response of the climate system to the anthropogenic forcing. Given the complexity of coupled processes in the climate system, this task will be a true challenge to climate researchers for many years to come.