Matin Durrani visits the National Institute of Standards and Technology in the US to find out how neutrons are being used to create a unique microscope that can see inside objects that other particles fail to reach
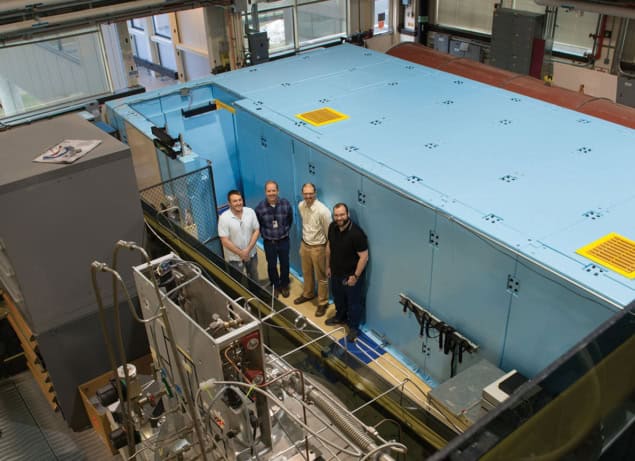
It’s not often that a police officer greets you when you enter a physics laboratory. But then the Center for Neutron Research (CNR) at the National Institute of Standards and Technology (NIST) in the US is no ordinary lab. Nestled amid the green lawns of NIST’s Gaithersburg campus just north of Washington, DC, it houses a 20 MW nuclear reactor – powered by highly enriched uranium – that first went critical in 1967. For added safety, the centre is surrounded by large boulders to prevent unauthorized vehicles from driving up too close.
Once you are past security though, the CNR looks and feels like any ordinary neutron-physics facility. Neutrons from the reactor are used by more than 2000 researchers from university, business and government for almost 200 days each year. Seven of the 28 experimental stations are diffractometers and spectrometers that use “thermal” neutrons that have been slowed by the reactor’s heavy water to energies of 15–20 meV. Researchers scatter these neutrons off materials to reveal their properties, to develop radiation detectors and to maintain dosimetry standards.
The other 21 instruments at the CNR are different. Located in a separate experimental hall, they sit at the end of guide tubes some tens of metres long. The neutrons that enter these tubes from the reactor are “cold”, having been slowed to energies of 5 meV or less after passing through a liquid hydrogen moderator. The inside walls of the guide tubes are coated with nickel, which has the highest total external reflection of any element. Cold neutrons that graze the walls at shallow angles therefore do not get absorbed but instead bounce off the inner surfaces, like stones skipping over a lake. Researchers use the cold neutrons for studying everything from polymers and proteins to magnets and non-Newtonian fluids.
One fascinating new project using cold neutrons at NIST involves building what the lab claims is the world’s most advanced “neutron microscope”. Like a medical X-ray of the human body, the intensity of the neutron “shadow” of an object provides information about its internal make-up. Water, for example, blocks neutrons whereas lead, aluminium and other dense materials let them through. So by comparing, say, the neutron image of a fuel cell containing water with images of the same object as it dries, researchers could study how water diffuses as the cell’s electrodes degrade. Other applications might include imaging lithium batteries, studying fluid flow in rocks or even analysing works of art.
Beyond the pinhole
NIST is not the only lab creating a neutron microscope, but its approach has certain advantages. Researchers at the Paul Scherrer Institute in Switzerland, for example, are building a neutron microscope by creating a detector with very high resolution. But to reach the resolution, incoming neutrons have to be sent through a tiny pinhole aperture to form a collimated beam that shines on a sample. The detector records the transmitted neutrons, which can then be analysed to yield information on the properties of the sample.
The problem is that in creating a collimated beam, few neutrons pass through the aperture in a given period, meaning that it can take a long time to image a sample. To take images faster or to improve the spatial resolution, your options are to either improve the detector, place the sample up close to the detector or use very thin samples. “But if you’re forced to have thin samples, you lose all the advantages of neutrons over X-rays,” says Dan Hussey, who leads NIST’s neutron-microscope project. “Even with better detectors, in a 1 μm pixel with a typical flux of 106 cm–2 s–1, there’s only one neutron every 100 seconds.”
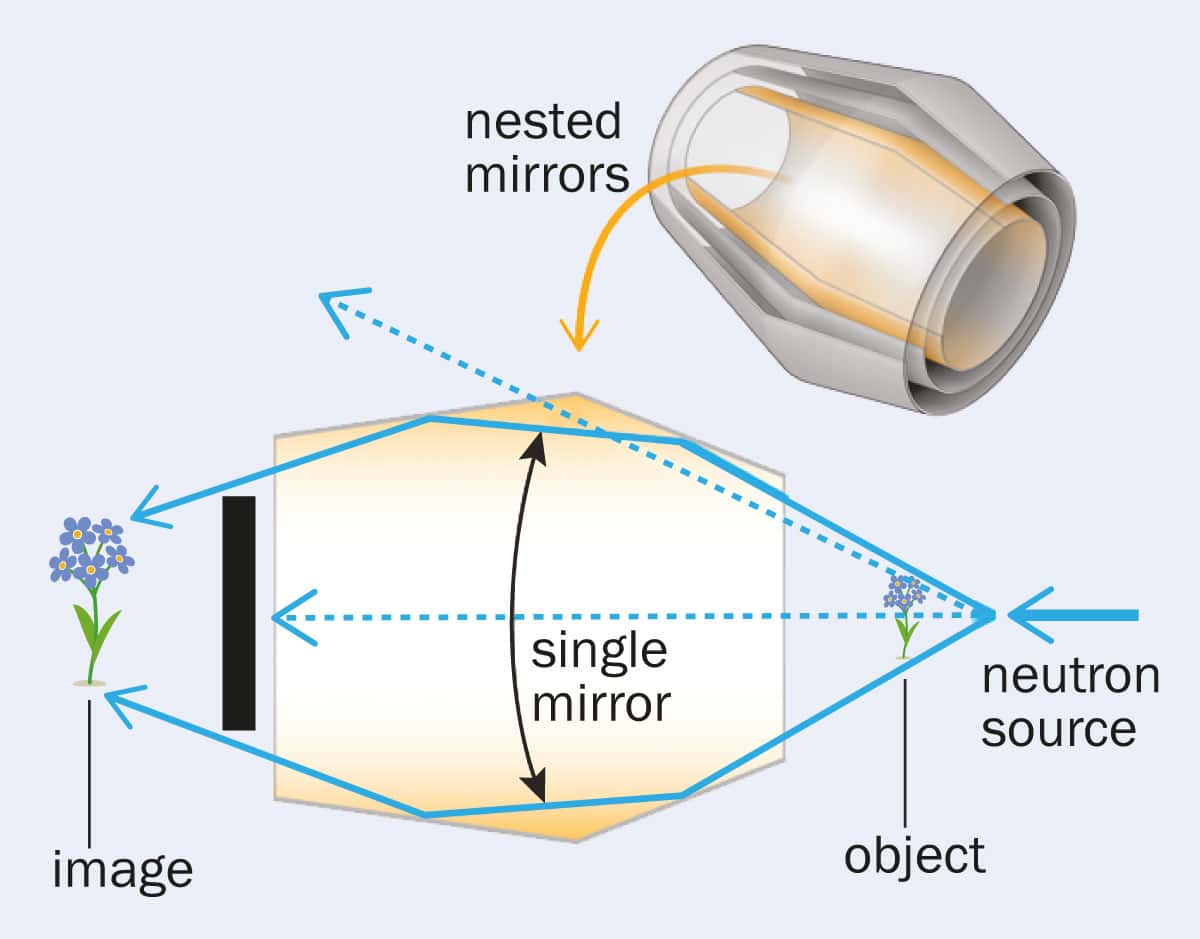
The neutron microscope at NIST instead does away with pinholes and uses a neutron lens. That might seem an odd approach given that neutrons have no charge and interact weakly with matter, meaning they can’t easily be focused into beams. NIST, however, is using a technique first proposed by German physicist Hans Wolter in 1952 to focus X-rays, which – like neutrons – are also hard to shepherd with conventional optics. “Wolter lenses” have been successfully used on various space-based X-ray missions, including NASA’s Chandra telescope and the European Space Agency’s XMM-Newton telescope.
In the NIST neutron microscope, neutrons from the lab’s reactor shine directly on a sample before passing through a Wolter lens and onto a detector. The barrel-shaped lens, which is roughly 20 cm long and 13 cm in diameter, consists of 10 nested parabolic mirrors each made of a 0.5 mm layer of highly polished nickel (see figure, right). Neutrons that hit a nickel cone at a shallow enough angle bounce off the inside and can be focused onto the sample. The neutrons then pass to a detector containing a 20 μm thick layer of gadolinium oxysulphide. Light given off when a neutron hits this material is recorded by a CMOS camera and fed to a computer for analysis.
Our target is to increase the beam intensity by a factor of 100 and achieve a spatial resolution of 1 μm
Dan Hussey
“The Wolter lenses mean that many more neutrons strike the sample than if there was a pinhole because you no longer need to collimate the beam,” says Hussey, who is developing the lenses with teams led by Mikhail Gubarev from NASA’s Marshall Space Flight Centre and Boris Khaykovich at the Massachusetts Institute of Technology. “Our target is to increase the beam intensity by a factor of 100 and achieve a spatial resolution of 1 μm.” Currently NIST’s best effort using conventional pinhole optics is just 15 μm.
Forging ahead
The 10 mirrors, which range in radius from 55 to 68 mm, are being made at NASA’s Marshall lab by electroplating nickel onto a specially shaped and polished aluminium block, and then peeling the metal off after it’s been cooled in an ice bath. NASA is still fine-tuning the manufacture process, which is not easy. Gravity makes the foils sag by about 80 nm and so they have to be carefully adjusted in the Wolter lens to avoid losing resolution. “However, the beauty of the method is that the mirrors can be replicated from substrates so if any other neutron lab had similar needs they could use the same substrates to create their own neutron microscope,” says Hussey.
The other advantage of using Wolter lenses is that the sample can be placed a long way from the detector – 7 m in the case of NIST – allowing researchers to obtain magnified images of the sample, with Hussey hoping for magnifications of at least ×10. With a pinhole camera, in contrast, the sample has to be stationed right up close to the detector to maximize neutron flux, meaning it is hardly magnified at all. When the Wolter lens is complete in 2018, Hussey says that rather than taking 20 minutes to capture an image – as with pinhole optics – it will take just 20 seconds or less.
NIST’s plan is for the neutron microscope to become a fully functional user facility in 2018. The lab also wants to improve the system to allow neutron phase imaging, which uses variations in the phase of the signal – not its attenuation – to determine the details of a material’s structure. As Hussey and colleagues put it in a paper demonstrating the principle of the Wolter lens for neutron microscopy in 2013 (Appl. Phys. Lett. 102 183508), their work could allow “game-changing improvements in the neutron-imaging technique”.