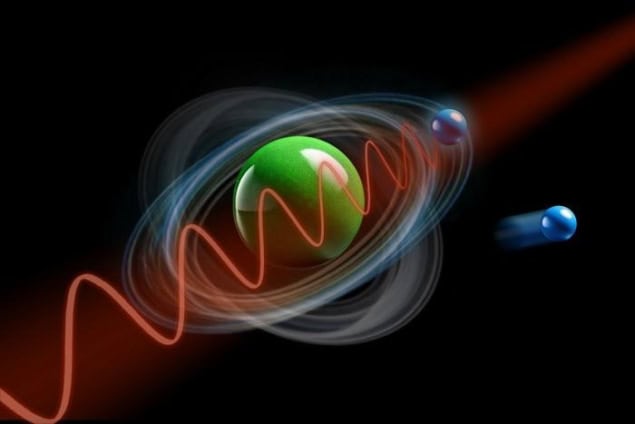
The effect of quantum entanglement on the emission time of photoelectrons has been calculated by physicists in China and Austria. Their result includes several counter-intuitive predictions that could be testable with improved free-electron lasers.
The photoelectric effect involves quantum particles of light (photons) interacting with electrons in atoms, molecules and solids. This can result in the emission of an electron (called a photoelectron), but only if the photon energy is greater than the binding energy of the electron.
“Typically when people calculate the photoelectric effect they assume it’s a very weak perturbation on an otherwise inert atom or solid surface and most of the time does not suffer anything from these other atoms or photons coming in,” explains Wei-Chao Jiang of Shenzhen University in China. In very intense radiation fields, however, the atom may simultaneously absorb multiple photons, and these can give rise to multiple emission pathways.
Jiang and colleagues have done a theoretical study of the ionization of a helium atom from its ground state by intense pulses of extreme ultraviolet (XUV) light. At sufficient photon intensities, there are two possible pathways by which a photoelectron can be produced. In the first, called direct single ionization, the photon in the ground state simply absorbs an electron and escapes the potential well. The second is a two-photon pathway called excitation ionization, in which both of the helium electrons absorb a photon from the same light pulse. One of them subsequently escapes, while the other remains in a higher energy level in the residual ion.
Distinct pathways
The two photoemission pathways are distinct, so making a measurement of the emitted electron reveals information about the state of the bound electron that was left behind. The light pulse therefore creates an entangled state in which the two electrons are described by the same quantum wavefunction. To better understand the system, the researchers modelled the emission time for an electron undergoing excitation ionization relative to an electron undergoing direct single ionization.
“The naïve expectation is that, if I have a process that takes two photons, that process will take longer than one where one photon does the whole thing,” says team member Joachim Burgdörfer of the Vienna University of Technology. What the researchers calculated, however, is that photoelectrons emitted by excitation ionization were most likely to be detected about 200 as earlier than photons detected by direct single ionization. This can be explained semi-classically by assuming that the photoionization event must precede the creation of the helium ion (He+) for the second excitation step to occur. Excitation ionization therefore requires earlier photoemission.
The researchers believe that, in principle, it should be possible to test their model using attosecond streaking or RABBITT (reconstruction of attosecond beating by interference of two-photon transitions). These are special types of pump-probe spectroscopy that can observe interactions at ultrashort timescales. “Naïve thinking would say that, using a 500 as pulse as a pump and a 10 fs pulse as a probe, there is no way you can get time resolution down to say, 10 as,” says Burgdörfer. “This is where recently developed techniques such as streaking or RABBITT come in. You no longer try to keep the pump and probe pulses apart, instead you want overlap between the pump and probe and you extract the time information from the phase information.”
Simulated streaking
The team also did numerical simulations of the expected streaking patterns at one energy and found that they were consistent with an analytical calculation based on their intuitive picture. “Within a theory paper, we can only check for mutual consistency,” says Burgdörfer.

How long does the photoelectric effect take?
The principal hurdle to actual experiments lies in generating the required XUV pulses. Pulses from high harmonic generation may not be sufficiently strong to excite the two-photon emission. Free electron laser pulses can be extremely high powered, but are prone to phase noise. However, the researchers note that entanglement between a photoelectron and an ion has been achieved recently at the FERMI free electron laser facility in Italy.
“Testing these predictions employing experimentally realizable pulse shapes should certainly be the next important step.” Burgdörfer says. Beyond this, the researchers intend to study entanglement in more complex systems such as multi-electron atoms or simple molecules.
Paul Corkum at Canada’s University of Ottawa is intrigued by the research. “If all we’re going to do with attosecond science is measure single electron processes, probably we understood them before, and it would be disappointing if we didn’t do something more,” he says. “It would be nice to learn about atoms, and this is beginning to go into an atom or at least its theory thereof.” He cautions, however, that “If you want to do an experiment this way, it is hard.”
The research is described in Physical Review Letters.