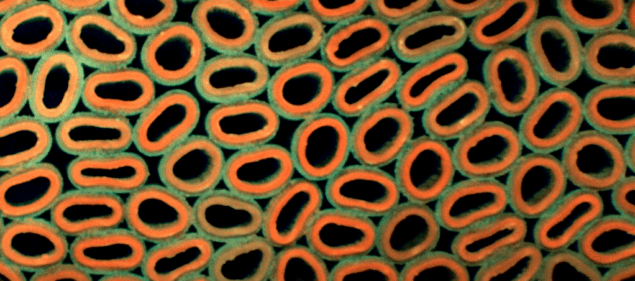
People working in industry, biology and geology are all keen to understand when particles will switch from flowing like fluids to jamming like solids. With rigid particles, and even for foams and emulsions, scientists know what determines this crunch point: it’s related to the number of contact points between particles. But for squishy particles – those that deform by more than 10% of their size – that’s not necessarily the case.
“You can have a particle that’s completely trapped between only two particles,” explains Samuel Poincloux, who studies the statistical and mechanical response of soft assemblies at Aoyama Gakuin University, Japan.
Factoring that level of deformability into existing theories would be fiendishly difficult. But with real-world scenarios – particularly in mechanobiology – coming to light that hinge on the flow or jamming of highly deformable particles, the lack of explanation was beginning to hurt. Poincloux and his University of Tokyo colleague Kazumasa Takeuchi therefore tried a different approach. Their “easy-to-do experiment” sheds fresh light on how squishy particles respond to external forces, leading to a new model that explains how such particles flow – and at what point they don’t.
Pinning down the differences
To demonstrate how things can change when particles can deform a lot, Takeuchi holds up a case containing hundreds of rigid photoelastic rings. When these rings are under stress, the polarization of light passing through them changes. “This shows how the force is propagating,” he says.
As he presses on the rings with a flat-ended rod, a pattern of radial lines centred at the bottom of the rod lights up. With rigid particles, he explains, chains of forces transmitted by these contact points conspire to fix the particles in place. The fewer the contact points, the fewer the chains of forces keeping them from moving. However, when particles can deform a lot, the contact areas are no longer points. Instead, they extend over a larger region of the ring’s surface. “We can already expect that something will be very different then,” he says.
The main ingredient in Takeuchi and Poincloux’s experimental study of these differences was a layer of deformable silicone rings 10 mm high, 1.5 mm thick and with a radius of 3.3 mm, laid out between two parallel surfaces. The choice of ring material and dimensions was key to ensuring the model reproduced relevant aspects of behaviour while remaining easy to manipulate and observe. To that end, they added an acrylic plate on top to stop the rings popping out under compression. “There’s a lot of elastic energy inside them,” says Poincloux, nodding wryly. “They go everywhere.”
By pressing on one of the parallel surfaces, the researchers compressed the rings (thereby adjusting their density) and added an oscillating shear force. To monitor the rings’ response, they used image analysis to note the position, shape, neighbours and contact lengths for each ring. As they reduced the shear force amplitude or increased the density, they observed a transition to solid-like behaviour in which the rings’ displacement under the shear force became reversible. This transition was also reflected in collective properties such as calculated loss and storage moduli.
Unexpectedly simple
Perhaps counterintuitively, regular patterns – crystallinity – emerged in the arrangement of the rings while the system was in a fluid phase but not in the solid phase. This and other surprising behaviours make the system hard to model analytically. However, Takeuchi emphasises that the theoretical criterion for switching between solid-like and fluid-like behaviour turned out to be quite simple. “This is something we really didn’t expect,” he says.
- The top row in the video depicts the fluid-like behaviour of the rings at low density. The bottom row depicts the solid-like behaviour of the rings at a higher density. (Courtesy: Poincloux and Takeuchi 2024)
The researchers’ experiments showed that for squishy particles, the number of contacts no longer matters much. Instead, it’s the size of the contact that’s important. “If you have very extended contact, then [squishy particles] can basically remain solid via the extension of contact, and that is possible only because of friction,” says Poincloux. “Without friction, they will almost always rearrange and lose their rigidity.”
Jonathan Bares, who studies granular matter at CNRS in the Université de Montpellier, France, but was not involved in this work, describes the model experiment as “remarkably elegant”. This kind of jamming state is, he says, “challenging to analyse both analytically and numerically, as it requires accounting for the intricate properties of the materials that make up the particles.” It is, he adds, “encouraging to see squishy grains gaining increasing attention in the study of granular materials”.
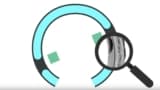
Fluids flow faster in liquid-walled channels
As for the likely impact of the result, biophysicist Christopher Chen, whose work at Boston University in the US focuses on adhesive, mechanical and biochemical contributions in tissue microfabrication, says the study “provides more evidence that the way in which soft particles interact may dominate how biological tissues control transitions in rigidity”. These transitions, he adds, “are important for many shape-changing processes during tissue assembly and formation”.
Full details of the experiment are reported in PNAS.