Researchers have long sought to observe fast-moving events at ever-higher speeds. Charalampos Tsoumpas explains why our ability to track gamma rays emitted just picoseconds apart could lead to a new generation of medical-imaging devices
I once watched a YouTube video that blew my mind. Created in 2011 by Ramesh Raskar and colleagues at the Massachusetts Institute of Technology (MIT) in the US, it showed a pulse of visible laser light less than one-trillionth of a second (10–12 s) long, travelling down an empty, horizontal plastic fizzy-drink bottle (see video below). The light bounced off the inside of the cap, creating a blinding flash as it did so.
Involving millions of repeated measurements, the filming technique was complicated, but it allowed the MIT group to create videos at an effective rate of a trillion frames per second. So although the light took just a billionth of a second (10–9 s) to travel down the bottle, you could see the movement and reflection of the pulse in all its glory. Obviously, to allow the event to be watched properly, the video was shown in super-slow motion, with the light’s journey lasting about 10 seconds when viewed online.
The video was just one of the latest attempts in the quest for “ultrafast photography”, an endeavour that began in 1878 with the recording of a galloping horse and was followed nine years later by the photograph of a bullet moving faster than the speed of sound. Decades later, the development of charge-coupled devices (CCDs) and then complementary metal-oxide-semiconductor (CMOS) technology let researchers study moving objects at rates of up to 107 frames per second. But the 2011 video showed that events could now be captured at a trillion (1012) frames per second.
The big snag with the MIT filming method was that the researchers had to repeat the experiment over and over again, gradually building up their animation of the light. Synchronizing the camera and the laser that generates the pulse – to ensure the timing of every exposure was the same – also needed lots of advanced optical kit and delicate mechanical control. Indeed, it took only a nanosecond for light to scatter through the bottle but an entire hour to collect all the data, prompting Raskar to joke that his system was “the world’s slowest fastest camera”.
However, in 2014 researchers at Washington University in St Louis, US, led by Liang Gao (now at the University of California), developed a way of directly imaging ultrafast events in a single go, rather than having to film them repeatedly. Their technique, dubbed “compressed ultrafast photography”, operates at rates up to 1011 frames per second (Nature 516 74). They used it to take a snapshot of a single photon, which meant they had video recorded the fastest physical entity in the universe (see video below).
Medical magic
With such ultrafast imaging techniques constantly evolving, researchers have naturally wondered about other potential applications, such as studying ultrafast phenomena in biological cells. But could we extend such techniques so they don’t just work with visible light (roughly 1 eV) but detect much higher-energy X-rays (0.1–100 keV) and gamma rays (>100 keV) too? Being able to track such photons with high temporal and spatial resolution would have a massive impact in medical physics.
High-energy photons are already vital in X-ray computed tomography (CT), positron emission tomography (PET) and single photon emission computed tomography. While some of these techniques simply take snapshots of structures within the body (think of a standard X-ray of a broken bone) others look at physiological functions such as blood flow, metabolism and chemical absorption. Being able to detect high-energy photons with higher spatial and temporal resolution would allow us to follow dynamic processes in the body even more closely and continuously.
Take PET, which can measure how fast glucose is consumed around the body, or provide information about blood flow and oxygen use. The technique involves injecting patients with a radioactive tracer, such as fluorine-18. Positrons emitted by the radioisotope collide with electrons in the patient’s tissue, annihilating each other to produce two gamma-ray photons with an energy of 511 keV. Travelling in opposite directions, they’re captured by “scintillator” crystals, triggering the release of lower-energy photons that a photodetector converts into an electric signal.
In revealing the distribution in time and space of the annihilation events, PET therefore indicates roughly where the radioisotope tracer has spread to at a particular time. That information is vital because it tells us how fast the tracer has been able to travel to and from various tissues and organs in the body. PET, in other words, helps us to understand biochemical processes in the body and distinguish between normal and abnormal behaviour.
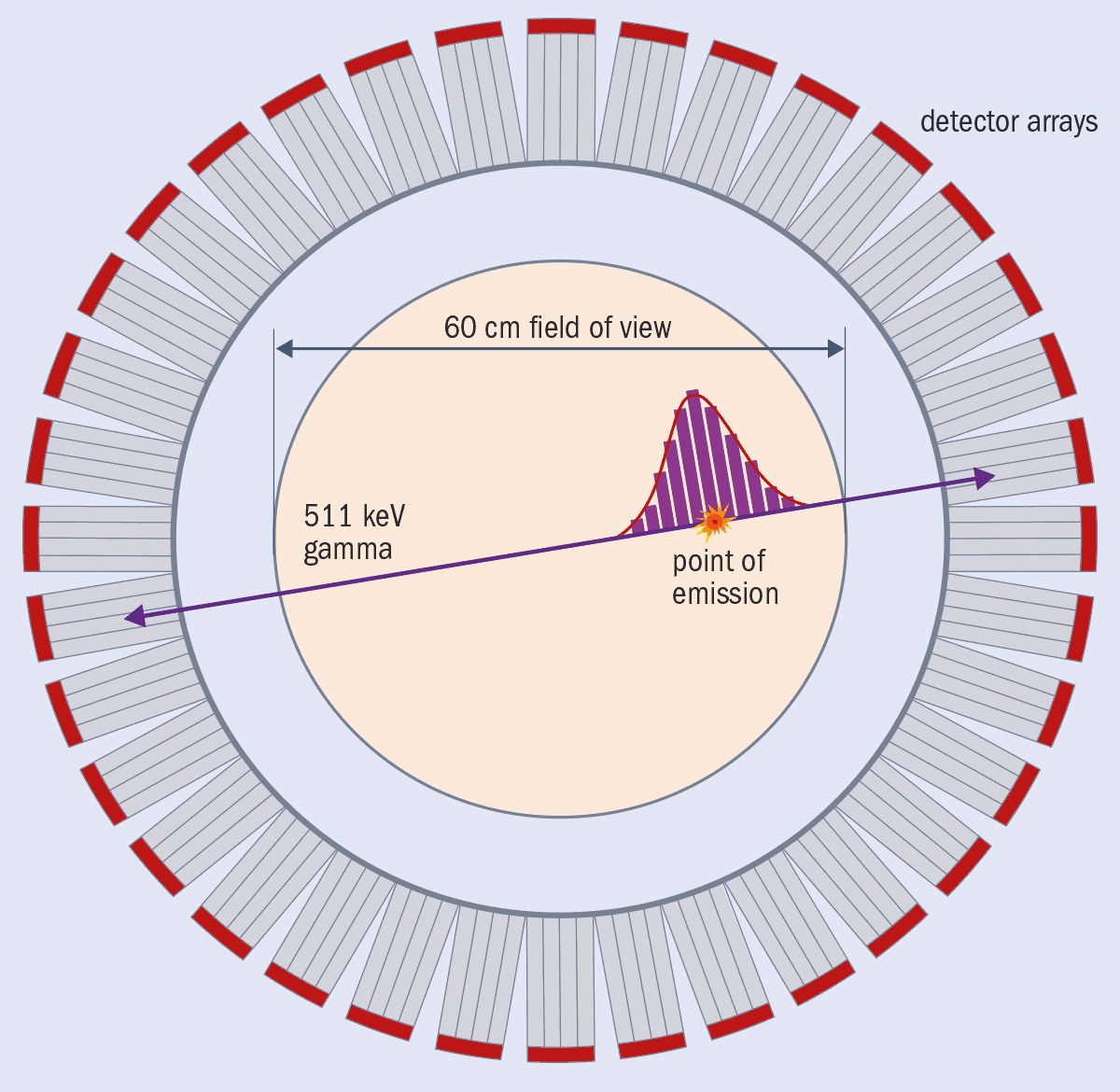
Today’s best state-of-the-art clinical PET scanners can achieve a resolution of about 3–4 mm in a scan that lasts a couple of minutes and they offer reasonably good (10%) energy resolution, thereby providing vital information about the biochemical behaviour of the radiotracer in the body. Better images are possible using a new variety of machine known as time-of-flight PET (TOF-PET) scanners, which can measure the tiny time difference between when the two annihilation photons are detected. The world’s current fastest TOF-PET commercial scanner, which is built by Siemens, can resolve processes down to just above 200 picoseconds (200 × 10–12 s) (figure 1).
That kind of timing resolution is excellent and, what’s more, the signal-to-noise ratio is 2.5 times higher than with PET scanners that don’t use timing information. So, if – and it’s a big if – detectors could operate at still faster speeds of, say, 10 ps, we’d get even better maps of where the photons originated. Indeed, the signal-to-noise ratio of the images is expected to rise by a factor of 12 according to a formula developed by Maurizio Conti and colleagues from Siemens in 2013 (IEEE Transactions on Nuclear Science 60 87).
Access all areas
Huge improvements of that kind, brought about by high-resolution, ultrafast gamma-ray detectors, could bring many practical benefits in medicine. Clinicians would be able to examine patients faster, reduce the isotope doses they need, and improve the quality, resolution, accuracy and precision of the images that are so vital for medical diagnoses. But such detectors could benefit many other areas too, which is what prompted the European Union (EU) to fund the Fast Advanced Scintillation Timing (FAST) project.
Led by Etiennette Auffray Hillemanns, a physicist at the CERN particle-physics lab near Geneva, FAST ran from 2014 to 2018. Also known as Action TD1401, this multidisciplinary project (of which I was communications manager) was part of the European Cooperation in Science and Technology (COST). Bringing together hundreds of academics and industrialists from 25 different nations, our aim was to create fast, scintillator-based detectors with sub-100 ps timing precision.
In medical physics, such fast photodectors could help develop TOF-CT scanners (Phys. Med. Biol. 65 085013), Cherenkov imaging and neutron imaging. In biology, they could be vital for high-throughput microscopy, time-gated optical tomography, fast cell sorting, and applications where you want to use very low levels of light so you don’t destroy a live sample. Such detectors could also benefit astronomy by boosting our attempts to image gamma-ray bursts and other cosmic sources of high-energy radiation. Future high-luminosity particle colliders – such as the upgrade to CERN’s Large Hadron Collider – could reap the reward too. At a more everyday level, fast detectors will help in automotive LIDAR (light detection and ranging), which is vital for autonomous, self-driving cars.
With so much potential, members of the FAST project decided to focus on three key areas. The first was to find materials that can produce light more quickly (and given that there are many different types of scintillating crystals, this was a big job). The second was to find ways to transport the light faster through the material to ensure it’s collected more quickly by the photodetector. This is a key issue because the crystal in a PET scanner usually has to be 2–3 cm long to stop enough high-energy photons on their journey to the photodetector and so get a big enough signal. Finally, and most importantly, we wanted to develop ways to detect the scintillation light faster and convert it more quickly into an electric signal, while still measuring the deposited energy of the high-energy photon with good precision.
In reaching this final goal, it’s worth noting that fast detectors have been made possible thanks to solid-state silicon photomultipliers, which have replaced conventional vacuum-based photomultiplier tubes as the best way of recording signals from scintillator crystals in PET scanners. Consisting of thousands of single-photon avalanche diodes (SPADs), silicon photomultipliers have continually smashed their time-resolution records. But as Stefan Gundacker from the University of Milano-Bicocca and colleagues at CERN showed last year (Phys. Med. Biol. 64 055012), if we could read these photomultipliers at high frequency, we’d be able to manipulate the signal from each diode and still extract timing and energy information with high resolution.
The key thing about the PET technique is that it produces many scintillation photons, but they don’t all appear at the same time. Silicon photomultipliers help by lowering the threshold between the signal and electronic noise, thereby letting you measure more accurately when the very first scintillation photons in the crystal are produced – and therefore boost the timing resolution. Indeed, the quest to improve PET and other medical-physics applications is what inspired the early developers of silicon photomultipliers in the first place.
In the case of scintillator crystals made from lutetium oxyorthosilicate doped with cerium and calcium (LSO:Ce:Ca), specially adapted front-end electronics can detect the difference in the arrival time of the two annihilation photons – the so-called “coincidence time resolution” (CTR) – of 98 ps for a 2 cm-thick crystal (the size you typically get in an ordinary PET scanner) and just 58 ps for a 3 mm-thick crystal. Even better, the high-frequency-readout means you can exploit another signal too – Cherenkov light emitted by scintillator electrons that have picked up energy from the 511 keV gamma-ray photons and so started moving at faster than the speed of light in the material. This light gives you a way of “time stamping” the arrival of the very first scintillation photons with high timing precision.
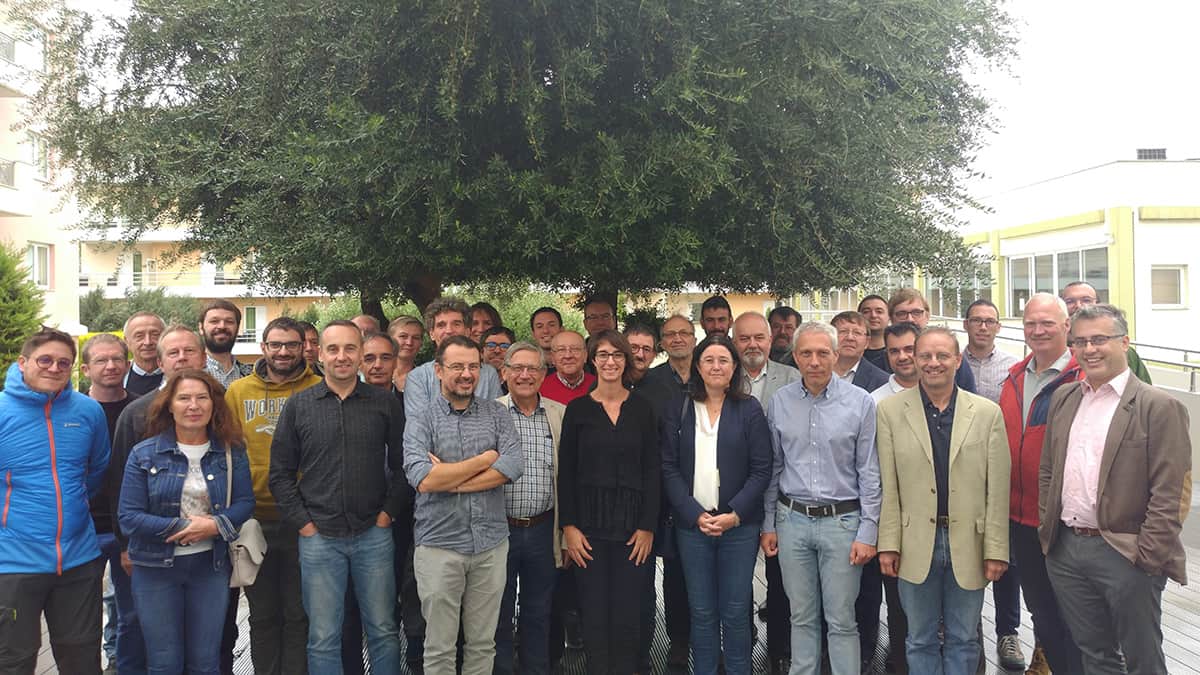
For another common PET scintillator – bismuth germatate (Bi4Ge3O12 or BGO) – the CTR was 277 ps for 2 cm-thick crystals and 158 ps for 3 mm crystals. These figures are interesting because although BGO appears slower for PET imaging, it is much cheaper to make than LSO. The other plus-point of BGO is that it’s denser than LSO, which means that a bigger fraction of the incident gamma-ray photons lose all their energy when they interact with the crystal, thereby strengthening the signal. The downside of BGO is that it’s got poor time resolution, so you can’t usually distinguish between events more than a few nanoseconds apart. But with the possibility of extracting timing information using Cherenkov radiation, we might see TOF-PET scanners fitted with BGO crystals at a lower cost.
Another way we could measure photons in PET scanners with a CTR approaching 10 ps would be to use direct-band-gap semiconductors that emit light from excitons (electron–hole pairs). One FAST project, for example, developed a material based on highly luminescent semiconducting nanocrystals made from zinc oxide and gallium embedded in a scintillating polystyrene matrix (Optics Express 24 15289). It emits lots of high-intensity light when the excitons in the polystyrene decay over sub-nanosecond times and then transfer their energy to the nanocrystals, which then scintillate. A second group, meanwhile, showed that a multiple-quantum-well heterostructure based on InGaN/GaN can result in ultrafast luminescence excitation rise and decay times within picoseconds (J. Luminescence 208 119).
Another promising scintillation mechanism for ultrafast timing is known as “hot intraband luminescence”, or IBL (J. Luminescence 198 260). It occurs when a high-energy photon deposits its energy in a scintillator, which within picoseconds spits out a handful of photons over a broad range of energies. Although the IBL yield is far too low for scintillators, it could possibly be combined with other fast-scintillation techniques to time-tag scintillation events and significantly improve time resolution. The IBL yield could also possibly be increased further by engineering the band structure of the material to boost the number and probability of allowed radiative intraband transitions.
In addition to investigating ultrafast scintillation processes, FAST investigated the key parameters for photodetectors and electronics to achieve a good timing resolution. For photodetectors, the photodetection efficiency and the single-photon response are essential to catch the scintillating photons with the fastest timing resolution from the SPADs and provide an electrical signal with the lowest timing jitter. The project also saw researchers develop electronics that could resolve events at picosecond time scales while also ensuring the detector used as little power as possible yet still having a large bandwidth and low noise.
Crystal-clear future
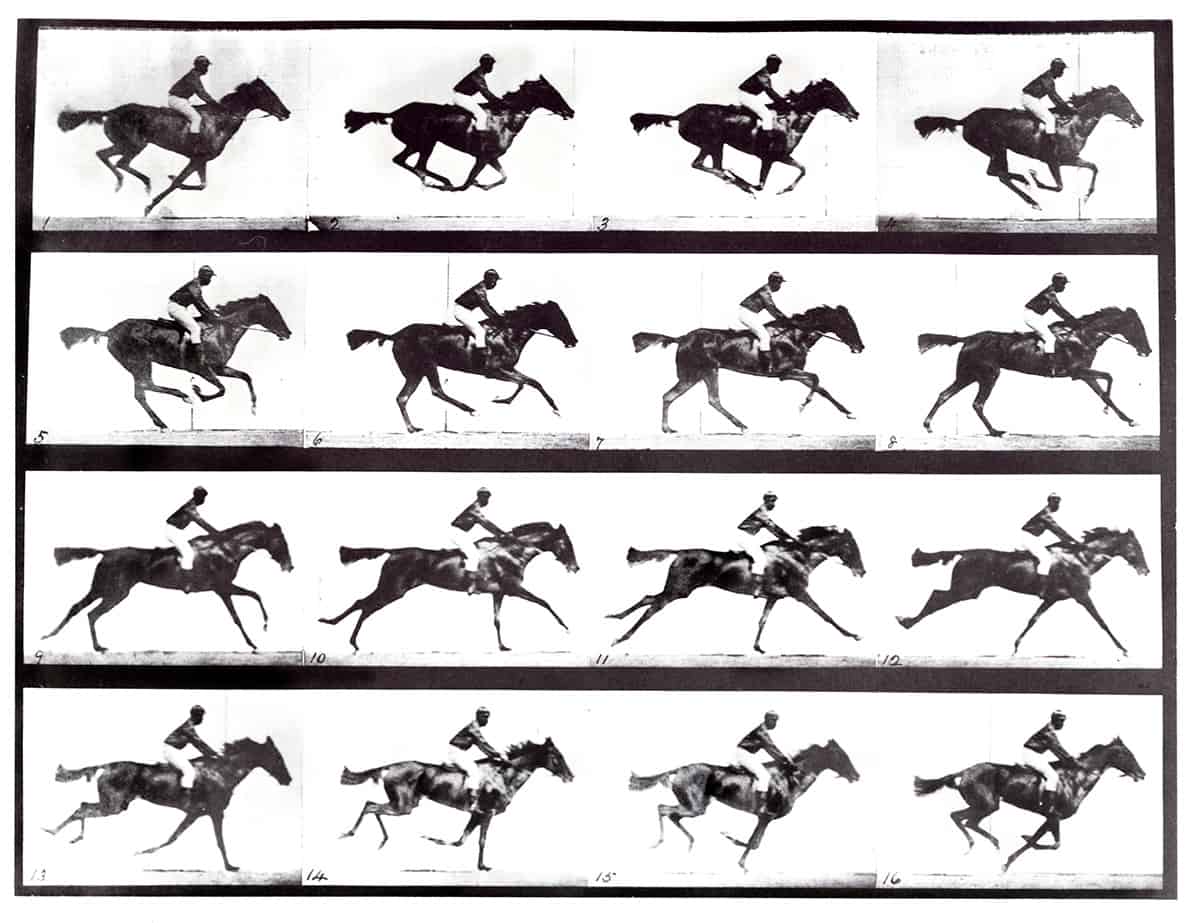
We’ve come a long way from that early 1878 recording of a galloping horse. Indeed, when it came to improving the timing precision of detectors, the FAST project exceeded our expectations. We showed it’s perfectly possible to achieve a CTR of 58 ps and indeed we are now targeting 10 ps timing levels, which would be invaluable in many areas of fundamental and applied science. I believe this objective may be demonstrated in the lab in the foreseeable future, though it’s hard to predict when we’ll see an entire PET scanner built from such fast high-energy photon detectors, let alone become financially viable. Still, I can’t wait to see the first images from an entire-body PET scanner operating with 10 ps CTR detectors (Med. Phys. 10.1002/mp.14122).
The EU FAST project ended in November 2018, but its activities are still alive via the Crystal Clear Collaboration, co-ordinated by researchers at CERN, which played a big role in FAST. Members of the collaboration have already launched a competition, challenging research teams to build the first detector that can resolve events with a time resolution of below 10 ps. Inspired by great historical contests of the past, such as the challenge to find a way to determine longitude at sea, this competition will, I hope, speed up the development of nuclear-instrumentation techniques and applications. And the more teams that join in, the likelier we are to succeed in this grand quest.